Last updated: January 25, 2018
Article
Monitoring Geothermal Systems and Hydrothermal Features
Cheryl Jaworowski
Duncan Foley
Heasler, H.P., Jaworowski, C., and Foley, D., 2009, Geothermal systems and monitoring hydrothermal features, in Young, R., and Norby, L., eds., Geological Monitoring: Boulder, Colorado, Geological Society of America, p. 105–140, doi: 10.1130/2009.monitoring(05). For permission to copy, contact editing@ geosociety.org. ©2009 The Geological Society of America. All rights reserved.
Introduction
Hot springs, geysers, mud pots, and fumaroles are dynamic surface features that represent interacting subterranean systems of water, heat, and rocks. Identifying the locations of these features and monitoring their heat, water flow, and chemistry can provide land managers with data needed to make informed decisions about management options.This chapter describes vital signs and contains options for monitoring surface and near-surface geothermal features, such as hot springs, geysers, mud pots, and fumaroles. Its focus is the description of techniques for detecting change in hydrothermal systems through time due to natural or human-related causes.
The goal of this chapter is to describe selected techniques for monitoring important vital signs of geothermal systems. Information in this chapter will not make the reader an expert in all geological aspects of geothermal systems. Some monitoring techniques are simple and can be performed by interested volunteers with no specialized background. Other techniques are complex and are best done by experts, with study results interpreted by seasoned practitioners. The described monitoring options may not meet all statutory and regulatory requirements that land managers may face.

However, there are some sites in the central and eastern states where thermal springs occur due to waters rising along deep faults or folded sedimentary rocks. Map from Green and Nix (2006).
The potential for geothermal systems exists over broad regions of the United States (Fig. 1). Although most of the geothermal systems occur in the western United States, isolated geothermal features are also found in the eastern United States.
The Geology of Geothermal Systems


At the surface, hot springs, geysers, mud pots, and fumaroles can form. Modified from Fournier et al. (1994).
by the infi ltration of rainwater or snowmelt. The system includes the underground flow of the waters, perhaps to depths of several kilometers, typically through fractures (Fig. 2) and/or pores in the underground rocks. It is important not to think of geothermal waters as typically flowing through underground caves (except in unusual circumstances). Water circulating near magma or deep enough to be heated by warm rocks forms hydrothermal fluids. Hydrothermal systems also include a zone where the hot waters flow upward to the land surface, driven by pressure or density, giving rise to a spring, geyser, mud pot, or fumarole. Figures 3 and 4 show geologic conceptions of how hydrothermal systems might look underground in areas where the heat source is volcanic and/or magmatic heat (Fig. 3) and where the heat source is non-magmatic heat (Fig. 4). In a magmatic hydrothermal system, the magma heats rocks that then heat deep circulating waters. In a non-magmatic hydrothermal system, thermal waters result from water being heated by higher temperature (through the increase in temperature with depth) rocks and quickly returned to Earth’s surface before cooling.
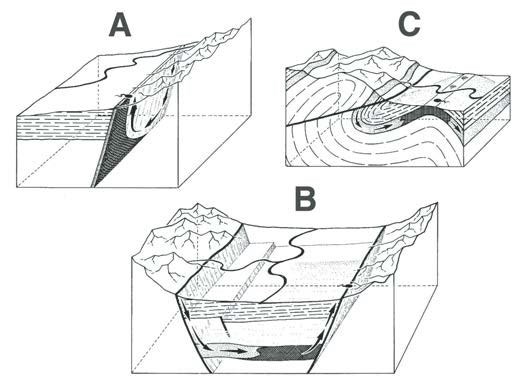
Drawing C shows a system where waters circulate to depth through rock units, and rise along the same units where they are folded upward. From Sorey et al. (1983).
expression of the hydrothermal system. Lack of active surface hydrothermal features may not mean, however, that the hydrothermal system ceased to exist. Perhaps the opening of new fractures redirected the flow of hydrothermal water, or perhaps mineralization sealed an existing fracture.
Changes from active to inactive and back again may be typical of hydrothermal systems (Moore, 2007). In Yellowstone, for example, forests have grown during periods of quiescence only to be killed when hydrothermal systems rejuvenate (Marler, 1956). Preliminary dating of a Yellowstone geyser suggests that it may have had periods of activity that were separated by perhaps thousands of years of quiescence (Foley, 2006). Monitoring hydrothermal systems may help determine whether the systems are heating up, cooling down, or remaining the same.
Multiple possible combinations of water sources, heat sources, subsurface rock types, chemical reactions and flowpaths lead to the development of many different kinds of surface hydrothermal features (Table 1). The multiple possible fates of waters, as shown on Figure 3, include such issues as: do the waters boil underground? Does the steam separate or stay with the waters as they rise? Do the waters just cool as they rise, without boiling? Do the waters mix with other subsurface waters, either other thermal waters or cooler groundwater? Table 1 summarizes major categories of hydrothermal systems.
Five closely related investigations of hydrothermal systems include: (1) identification, (2) monitoring, (3) research, (4) exploration, and (5) production. Identification is simply locating the surface expression of a geothermal feature and perhaps noting its temperature and flow. Monitoring, for the purposes of this chapter, is the short-term or long-term observation of the thermal, chemical, or flow characteristics of a surface or near-surface (for example, cave or well) thermal feature or connected group or groups of features. Monitoring also may involve describing how hydrothermal systems change through time and as a result of human activities. Research about hydrothermal systems may include more detailed thermal studies, in-depth chemical analyses, geophysical studies of electrical, magnetic, gravity, and seismic characteristics of a site or region, and detailed flow monitoring. Research also may include investigation of the entire hydrothermal system, rather than just surface phenomena. Exploration for geothermal resources builds on research and tries to find commercially producible quantities of hydrothermal fluids for either direct heat use (e.g., space heating or industrial processing) or for the generation of electricity. Production of a geothermal resource, once the resource is developed by extensive drilling, requires continual studies to assure that adequate quantities of fluids with the necessary temperatures and pressures are present in the system.
In this chapter, the emphasis is on monitoring vital signs of surface or near-surface phenomena in hydrothermal systems to assess natural and human-related changes. It does not include description of techniques that can be used for deep exploration or for monitoring production from a geothermal field. Nor does this chapter include discussion of biological monitoring of hydrothermal systems or monitoring underwater hydrothermal features. While monitoring these may be important in some cases, the sophistication of required equipment and personnel places such work still in the realm of research. Also, enhanced geothermal systems, which are created by drilling two wells into hot but not necessarily permeable rocks, artificially creating or enhancing natural permeability, and circulating water between the wells, are not part of this chapter. Thermal waters produced with petroleum products are also not discussed. In some cases, it may be important for land managers to consider these other thermal systems and consult with professionals.
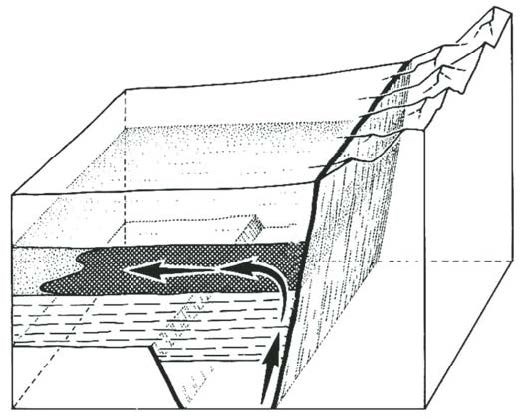
The geothermal industry and the U.S. Geological Survey divide hydrothermal systems into two subclasses based on chemically determined maximum subsurface fluid temperatures: (1) high temperature fluids, which are generally defined as those fluids above 90 °C (~195 °F) and may be suitable for the generation of electricity, and (2) low and moderate temperature fluids, which are those that have likely maximum subsurface fluid temperatures below 90 °C (~195 °F). Moderate and low-temperature fluids may be suitable for direct use, such as industrial drying, aquaculture, and space heating. It is worth noting that not all high temperature systems relate to volcanoes. For example, there is no volcanic activity around the Dixie Valley hydrothermal power plant in Nevada (McKenna and Blackwell, 2004; Kennedy and van Soest, 2006).
Hydrothermal systems exist in a continuum of resource temperatures. At the lowest end, geothermal resources grade into normal-temperature resources. In most non-geothermal areas, the temperature of shallow groundwater will be approximately the same as the mean annual air temperature. With heat pumps, almost all areas of the United States can derive useful heat from normal temperature groundwater. Monitoring normal temperature groundwater is not covered in this chapter.
Why Monitor Hydrothermal Systems?
Hydrothermal systems can change as a result of local or distant events that alter the water source or flow path, the heat source, thermal characteristics along the subsurface flow path, and/or the fractured rock that the waters are flowing through. Some of these changes may be natural, for instance as a result of weather patterns, climate changes, or earthquake activity. Other changes may relate to human activities, such as nearby production of petroleum resources, geothermal energy (Sorey, 2000) or even pumping a water well. Changes in flow may be either rapid, for instance as a result of an earthquake, or slow, as water supply changes with climate. Table 2 lists some reasons to monitor hydrothermal systems.
Stressors/Possible Change
Geothermal heat is the fundamental driver of hydrothermal systems. But, hydrothermal systems also require water and permeability. Changes in water quantity, changes in heat source, changes in permeability, and human activities interact to alter hydrothermal systems.

Many different geologic processes can bring about changes in these stressors. Changes in water quantity, for example, may occur as climate changes or new patterns of precipitation develop. Changes in the heat source can occur as a result of either an input of new magma or cooling of magma. Changes in permeability can occur as minerals precipitate along fractures and fill pore space and as earthquakes open or close fractures. Human activities may noticeably change water quantity through pumping nearby wells or otherwise removing or adding water to hydrothermal systems.
Because hydrothermal systems are linked by interactions of water systems, heat systems, and rock systems, they can provide monitoring data useful to many geologic disciplines. Hydrothermal systems can provide early evidence of moving magma (see Smith et al., this volume), potential hydrothermal explosions (Fournier et al., 1991), or even earthquakes (Silver and Valette-Silver, 1992).
GENERAL SAFETY DISCUSSION
(B) Thermal-infrared photograph of the same area shown in A. The area between the steam vents and the trees is hot (50-75 °C) and extremely dangerous.
Hydrothermal features and areas may experience rapid, drastic changes. Field teams must be aware of changing conditions at all times. Also, field personnel should carefully reevaluate safety each time they visit a thermal feature or thermal area. Because conditions may have changed since the last visit, a formerly safe approach may turn deadly.
Toxic gases may be present in hydrothermal areas. Depending on the heat source for the hydrothermal system, gases that may exist in dangerous concentrations include sulfur dioxide, hydrogen sulfide, and carbon dioxide. Field personnel need to be aware of the potential for the presence of such gasses and implement appropriate safety procedures.
It is important that the field team is aware of safety concerns before entering a thermal area. Pre-visit safety awareness may include literature reviews of the chemistry, literature reviews of hydrothermal site conditions, and discussions with experienced personnel. With each visit to a hydrothermal area, the field team should conduct a careful evaluation of any changes in the hydrothermal system that may affect safety.
VITAL SIGNS OF HYDROTHERMAL SYSTEMS
Vital Sign 1: Location of Hydrothermal Features
Locating hydrothermal features is the most basic way to describe and identify hydrothermal features. Names of individual features may change over time, whereas their geographic coordinates are unique. Coordinates may include the familiar latitude and longitude grid system, Universal Transverse Mercator (UTM), or State Plane Coordinate System (SPCS). The Public Land Survey System (PLSS) is another common method used to locate features on a map.
Locating hydrothermal features is a key component of mapping and the first step in monitoring hydrothermal features. When located and mapped, relationships to rivers, infrastructure, other hydrothermal features, and landmarks are apparent. Documenting and monitoring the location of hydrothermal features as well as other attributes allows a database of temperature, chemistry, and photography to be constructed. Digital archives of hydrothermal features assists information sharing, change detection, and integration with other spatial data.
Level One: Basic Mapping and Repeat Photography
Methods. The simplest way to map the location of a hydrothermal feature is to place the feature on a topographic map. Knowledge of basic map reading skills such as topographic contours, landforms, coordinate systems, and map scale allows personnel to plot the location of the thermal feature on a map (refer to Case Study I).
Topographic maps may display several coordinate systems: geographic coordinates (latitude, longitude), UTM coordinates (eastings and northings), and SPCS (x, y). Latitude and longitude are angular distances of measure (spherical coordinates) and difficult to use when plotting a location in the field. In contrast, the UTM coordinate system uses a linear scale of measurement based on dividing the Earth into 60 zones and measuring locations in meters from baselines established for each zone. This linear measurement of distance means that field personnel can use a simple scale, ruler, or grid to easily and consistently plot hydrothermal features on a map or determine coordinates of a new hydrothermal feature.
When reporting the coordinates, field personnel should note the datum of the coordinate system along with its x, y coordinates (i.e., latitude and longitude or northings and eastings). Datums are a set of parameters and control points used to accurately define the three-dimensional shape of the Earth. U.S. Geological Survey topographic maps commonly use the North American Datum (i.e., NAD 27). Incorrect datums or no information about the datum cause position errors (tens of meters) and negate efforts to accurately map a location. Coordinates (x, y, and the datum) can be entered into a spreadsheet along with elevation, date of observation, name(s) of field personnel, and name (if any) of the hydrothermal feature.
Repeat photography may show changes in morphology of the hydrothermal feature, changes in the outflow channel (amount of water, color, shape), or changes in the microbial community at a variety of scales. Changes in vegetation, either new vegetation growing or established vegetation being killed, may be identified. The challenge is to gather enough information to document natural changes and distinguish natural changes from anthropogenic changes.
When locating a hydrothermal feature, it is wise to determine a site for repeat photography. Characteristics of a good site for repeat photography include a clear view of the hydrothermal feature as well as its spatial extent, identifiable landmarks, and accessibility to the site. Information about the photographs can be added to a spreadsheet. In addition to repeat photography, this spreadsheet becomes a central data repository for temperature
measurements and chemical measurements of hydrothermal features.
Visualization and analyses of the spreadsheet data is important for understanding hydrothermal systems. It is difficult to see relationships between attributes of hydrothermal features or temporal trends within a spreadsheet or table. When viewed as a graph or map, the same data easily allows spatial relationships and temporal trends to be discerned. A variety of free to lowcost software allows visualization of spreadsheet data and three dimensional viewing of map information.
Equipment and costs. The easiest map to use for documenting the location of hydrothermal features is a U.S. Geological Survey 7.5 minute topographic map. Topographic maps display elevation, contours, streams, lakes, infrastructure, coordinate systems, and other cartographic information. These 1:24,000 scale topographic maps cover an area of ~10 by 14 km and are available at no cost on the Internet from the U.S. Geological Survey. Scales designed for consistently reading map coordinates (lat/long or northings and eastings) are available for less than $20 (all amounts herein are in US$). Waterproof notebooks and all-weather pens preserve field data, especially when field personnel are working during wet weather. Although any existing camera allows repeat photography, a digital camera is excellent. Digital images allow links in databases, easy comparisons with other digital photographs, and information sharing. A computer capable of storing electronic images and entering data into a spreadsheet program is necessary.
Limitations. Limitations include the spatial accuracy of the location and the need for data storage and/or archival space. The most significant limitation is the accuracy of locating hydrothermal features on a topographic map. Factors affecting spatial accuracy include the inherent accuracy of the topographic map, identifiable landmarks, changes in topography, vegetation changes, date of the topographic map, and skills of field personnel. Digital photographs require space for data storage and space for backup and/or archive of images.
Interpretation. The estimated precision of a hydrothermal feature’s location affects how changes in the location of the hydrothermal feature are evaluated. For example, if the hydrothermal feature is poorly located (within 10 m), then it is difficult to detect small changes. Level-one mapping can easily document changes in hydrothermal feature location or activity if new hydrothermal features appear or existing features cease being active.
Level Two: GPS Survey and Monuments for Repeat Photography
Methods. A handheld, recreational-grade global positioning system (GPS) receiver can improve the accuracy and precision of locating hydrothermal features. With a handheld GPS receiver, field personnel directly gather coordinates in the field. Field personnel need to consider their personal safety when approaching a hydrothermal feature and should note the GPS position with respect to the hydrothermal feature (i.e., coordinates were taken at the northern edge of a 10-m-wide pool). Although GPS receivers and associated data loggers automatically log positions, field personnel also should write the feature’s x-y coordinates and associated map datum in a waterproof field notebook. This recommendation is important whether field personnel are taking measurements in easily accessible hydrothermal areas or difficult-to-access hydrothermal areas. The precision of the GPS receiver determines the number of hydrothermal features to be mapped or located within an area. For example, if the GPS receiver is precise to ±4 m, it makes little sense to map hydrothermal features that are 2 m apart.
For repeat photography, a high-quality camera, fixed photographic monuments, and photographic software improve the accuracy of locating hydrothermal features and detecting change within the hydrothermal system. Some digital cameras allow input from a GPS receiver, thereby locating the site of the photograph. The GPS data become part of the data file that accompanies each photograph. A fixed photographic monument can be as simple as a mark on a handrail or the ground. With fixed photographic monuments, field personnel can take photographs from a consistent location. With a fixed location, personnel can view time-sequences of digital photographs and may see change occurring over days, weeks, months, or years. Documentation of change is more exact due to the improved estimate of location and establishment of photographic monuments.
Visualization and analyses of data are important at any level. With moderate funds, data from the GPS receiver can be integrated with low-cost software for comparison of photographs, low-cost geographic information system (GIS) software or any existing GIS programs. Professional versions of visualization software allow users more options for importing GIS data and visualizing the data with existing or newly acquired high-spatial resolution airborne imagery. Some photographic hardware and software even allow the creation of a movie from photographs taken from the same location through time.
Timing and frequency. Similar to level-one efforts, the dynamic and changing hydrothermal activity ultimately determines the timing and frequency of additional mapping and repeat photography. The types of hydrothermal systems (stable silica-rich, hot spring systems, acidic hydrothermal areas, rapidly depositing, carbonate-rich systems, hydrothermal explosions or collapse features) also influence whether visits are annual, monthly, weekly, or daily. With a recreational-grade GPS receiver, improved mapping precision may warrant more frequent visits by field personnel.
Equipment and costs. A recreational-grade, handheld GPS receiver costs hundreds of dollars. Basic digital cameras with associated software also cost hundreds of dollars, whereas specialized photographic software may cost thousands of dollars. Training for field personnel and field personnel time are additional costs.
Limitations. On-the-job training, increased data storage, and misrepresentations of hydrothermal features are limitations of the level-two survey. If field personnel are not already familiar with GPS technology, coordinate systems or low-cost digital mapping, then some on-the-job training is necessary for accurately locating hydrothermal features. Both level-one and level-two surveys of hydrothermal features view hydrothermal features as points on Earth’s surface. A point is a very simplistic way to represent a large irregularly shaped hydrothermal pool. Another limitation is the difficulty in determining coordinates for hydrothermal features that are closer to each other than the precision of the GPS receiver. The amount of data storage signifi cantly increases if a large megapixel digital camera is used for repeat photography.
Interpretation. Similar to level one, the estimated precision of a hydrothermal feature’s location affects how changes in the location of the hydrothermal feature are evaluated. For example, if the hydrothermal feature is only located to 5 m, it is difficult to detect small changes. However, the increased mapping precision of a level-two survey allows better documentation of changing hydrothermal activity.
Level Three: Mapping or Survey-Grade GPS Receivers and High-Spatial Resolution Airborne or Satellite Imagery
Methods. High-precision mapping or survey-grade GPS receivers accurately locate hydrothermal features. Mapping grade GPS receivers are precise to within ±20 cm using postprocessing differential corrections or real-time beacons. Survey grade GPS receivers identify locations within ±1 cm or less. A single point may represent an individual hydrothermal feature or a polygon may represent the perimeter and area of a hydrothermal pool. With personal safety in mind, field personnel can use distance-measuring lasers linked with the GPS receiver to create an offset distance and safely walk the perimeter around an extensive hydrothermal feature such as a pool. Additionally, high-precision GPS receivers potentially enable field personnel to map closely spaced hydrothermal features.
High-spatial resolution airborne (less than 1 m pixels) or satellite imagery (1–5 m pixels) allow efficient and safe mapping of hydrothermal features over a large area. Instrument platforms may include a balloon, an unmanned aerial vehicle (UAV), helicopter, a fixed-wing aircraft, or satellite. Airborne sensors may be visible and near-infrared. Digital cameras attached to a balloon can safely and efficiently map hydrothermal features within centimeters of their actual location (Planer-Friedrich et al., 2008). The precise location of hydrothermal features depends upon several factors, including (1) the pixel resolution of the camera or sensors, and (2) the accuracy of rectifying high-spatial resolution imagery to known locations on Earth’s surface. Image processing and GIS programs will import high spatial resolution imagery for georectification, display, analysis, and integration with other spatial information. At level three, visualization, mapping, image processing, GIS and GPS software reach the pinnacle of data integration. Software will correct field GPS coordinates, import real-time corrected coordinates from a field computer, and display the GPS data with existing GIS data or imagery for further analysis and data integration.
Timing and frequency. The cost and complexity of these level-three methods generally limits the mapping and monitoring of hydrothermal features to an annual effort. An optimal time for mapping hydrothermal features is late fall or when hydrothermal flow conditions are least affected by seasonal snowmelt or other events. During spring, seasonal snowmelt may mask hydrothermal waters. During winter, areas with little or no snow can
delineate active hydrothermal areas (Watson et al., 2008; White, 1969). Although mapping hydrothermal features during winter is possible, logistically it can be more difficult, especially if field personnel need to be deployed to support new acquisition of high-spatial resolution imagery.
Equipment and costs. Mapping- and survey-grade GPS systems (receivers, antennas, handheld computers, real-timebeacons, etc.) are available from a range of vendors. Mapping grade GPS systems cost thousands of dollars; survey-grade GPS systems cost tens of thousands. Software for collecting and correcting GPS data also needs to be purchased and installed on mobile mapping units and office computers. There is additional cost for software on portable mapping units and office computers. Field and office computer costs range from thousands to tens of thousands of dollars. Budgets should include: (1) additional software costs if the integration of GPS data with existing or new GIS data is desired; (2) a high-end computer with data storage, data back-ups, and archive capability for the high volume of data; and (3) a database manager. Additional costs may include maintenance for hardware and software, as well as technical support. Image acquisition costs vary considerably. Helium balloon equipment can be assembled for thousands of dollars. UAV and sensors are becoming more common and are available from a variety of commercial, government, amateur, and other sources. Under contract or a cooperative agreement, commercial companies, universities, and government agencies can fly hydrothermal areas and deliver oriented, georectified, and GIS-ready image mosaics. High-spatial resolution satellite imagery may cost thousands of dollars. The cost of software for processing, display, and analysis of images can vary from thousands to tens of thousands of dollars. If budgets preclude new image acquisition, then personnel may use existing high-spatial resolution (i.e., historic) aerial photographs (Shean, 2006) for mapping the location of hydrothermal features and detecting changes in hydrothermal activity.
Limitations. Equipment costs, highly skilled personnel, and time for surveys limit the frequency of high-precision GPS surveys of hydrothermal features. Even though the GPS receiver can locate hydrothermal features very precisely, field personnel may not be able to safely access some hydrothermal features. In addition to specialized software, highly trained personnel are necessary to acquire and process high-accuracy GPS locations.
Deveral limitations affect the use of high-spatial resolution imagery for locating hydrothermal features. These limitations include weather, accurate ground control points, equipment costs, image acquisition costs, image processing costs, and skilled personnel. Stable weather is necessary for handling a helium balloon and for acquiring airborne imagery. Accurate ground control points may be necessary for rectifying high-spatial resolution imagery to known locations on Earth’s surface. Highly trained personnel must insure that image acquisition, geometric corrections, and processed imagery meet the needs of the hydrothermal feature survey. Specialized image processing software is needed or the display, accurate rectifi cation of individual airborne images into a mosaic, and analysis of airborne imagery. Generation of a high-spatial resolution aerial mosaic is a time-intensive process for large areas.
Interpretation. Similar to level two, the estimated precision of a hydrothermal feature’s location affects how changes in the location of the hydrothermal feature are evaluated. Mapping and survey-grade GPS receivers locate hydrothermal features within centimeters. Balloon surveys also may result in centimeter precision. The accuracy and precision of locations derived from airborne imagery can vary considerably. Factors affecting the accuracy and precision include quality of ground control points, spatial resolution of the airborne imagery, and method of georectification.
Heat is a fundamental physical property of all geothermal systems. Heat is a form of energy that flows from high to low temperatures. Scientific units of measurement are the joule (Newton-meter) or calorie (energy required to raise 1 g of water from 14.4 °C to 15.4 °C). Scientific units of temperature measurement are in degrees Celsius. Temperature does not directly measure the total heat of a hydrothermal feature or area. The amount of heat emitted by a hot object is related to the temperature difference between an object and its surroundings, the mass of the hot object, and its specific heat. Thus, a thermal pool with a large mass and low temperature may emit more heat than a smaller pool with a small mass and high temperature.
Measured changes in temperature of a geothermal system may relate to the heat source of the geothermal system, heat transfer mechanisms within the system, and/or atmospheric conditions. Heat sources for a geothermal system can vary from molten rock to the normal temperature increase with depth below Earth’s surface (geothermal gradient). Heat transfer mechanisms include conduction, convection and radiation. Atmospheric conditions such as air temperature, high winds, and recent local precipitation can lower the surface temperature of a hydrothermal area. Ultimately, observed changes in temperature relate to the natural variability of geothermal systems (heat source or heat transfer mechanisms) and/or anthropogenic influences.
Temperature is an easy-to-measure physical parameter that characterizes a hydrothermal system. Low-cost, alcohol-bulb thermometers, digital temperature probes, and small handheld thermal-infrared (TIR) sensors all measure single point temperatures of thermal water or ground. The scientific goal of a geothermal monitoring plan defines the required precision and accuracy of the temperature measurement. For example, bulb thermometers generally are precise to within ±1–2 °C, whereas digital thermometers are precise to within ±0.1–0.01 °C. Immersion of the temperature-sensing portion of the thermometer in the same location is necessary for accurate and precise repeat temperature measurements (Figs. 8A and 8B). Representative temperatures of thermal features are best measured at or near the vents of the thermal features. Safety considerations sometimes necessitate the measurement of temperatures in outflow channels.
Water, rocks, sediments, and vegetation all emit thermal energy differently. This difference is an important parameter to know when estimating temperature with handheld TIR sensors and aircraft-mounted TIR detectors. Emissivity is a measure of how well objects radiate or emit thermal energy. Hot objects that emit 100% of their thermal energy at a specified temperature are perfect emitters of radiant thermal energy. Objects that emit less than 100% of their thermal energy are not perfect emitters of hermal energy. Perfect emitters of thermal energy would have an emissivity of 1.0. All other objects have an emissivity less than 1.0. If a handheld TIR scanner has a set emissivity of 1.0, then the temperature of a hydrothermal pool will be underestimated. For example, a thermal pool measured with a radiant measured temperature of 73 °C, emissivity 1.0, has a temperature closer to 76 °C, using an emissivity of 0.93 for water.
It is important to check the accuracy of temperature probes occasionally. A simple way to calibrate is to insert the probe into a vigorously stirred container of ice and water. The temperature of this mixture (the melting point of water) should be 0.0 °C at one atmosphere. If the measured temperature is significantly different from 0.0 °C, then the temperature probe should be recalibrated according to the manufacturer’s instructions. Most manufacturers will perform calibrations on temperature probes for an additional fee.
Level One: Single Point Temperature Measurements
Methods. The purpose of the geothermal monitoring program determines the precision, accuracy, and type of temperature sensors used. The goals of many geothermal monitoring efforts can be met using simple, handheld temperature probes. These simple temperature probes are precise to within a few tenths of a degree Celsius. Personnel should note the type and manufacturer of temperature sensing equipment used and record the type of sensing equipment in a waterproof fi eld notebook, electronic spreadsheet, or database.
When using an immersion temperature probe, field personnel should place the probe at the same location in the outflow channel for repeatability of temperature measurements.
Low-cost, handheld TIR temperature detectors have distinct advantages and disadvantages when compared to bulb and digital thermometers. Because field personnel do not have to immerse a probe into the thermal fluid, handheld TIR detectors are safer to use than immersion probes. The operator simply aims the TIR device at the spot to be measured and reads a temperature from the TIR instrument. Field personnel can measure many temperatures quickly and safely at different locations. However, the TIR sensor is only measuring the surface (skin) temperature of a hydrothermal feature or area of thermal ground. The temperature of the upper few microns may not be representative of the entire feature or area. Any vapor or steam rising from the hydrothermal feature or thermal area will impede the TIR instrument’s ability to measure the actual temperature of the hydrothermal feature.
The temperature being measured using TIR devices results from radiant heat transfer. Generally, radiant and immersion temperatures compare well, but sometimes may vary due to an object’s physical characteristics (see previous discussion on emissivity). Another disadvantage of handheld TIR sensors is that the temperature of an area is measured, not just the temperature of a single point. Field personnel must know the dimensions of the area being measured by the TIR sensor so that temperature measurements are made for the area of interest.
Field personnel should record all data in a waterproof field note book with an all-weather pen, then enter the data into a spreadsheet or database. Recorded data should include: date, time, coordinates of measurement, name of field personnel, type of temperature equipment, temperature, fluctuations of temperature, and any observations. Location and photographic documentation of the measurement site is important for consistency and
comparison with future temperature measurements.
Timing and frequency. Two key factors affecting timing and frequency are study design and accessibility. Once a temperature baseline has been established for a hydrothermal feature, the frequency of temperature measurements can decrease. The study design of a geothermal monitoring plan determines baseline data and maps. For example, a remote backcountry hydrothermal feature unaffected by human activities may only require annual monitoring. However, a similar hydrothermal feature affected by human activities may require monthly or weekly monitoring. Also, simple measuring techniques may not monitor remote hydrothermal features or areas adequately. Monitoring remote hydrothermal features may require temperature loggers. (See discussion in Vital Sign 3, level two, concerning temperature data loggers.)
When starting a monitoring program, temperature measurements should be made frequently to define any daily or seasonal variations. For a high-temperature hydrothermal feature with considerable water flow, the hydrothermal feature’s temperature may not vary significantly over time. Thus, one measurement every six months may be sufficient. External factors such as air temperature may affect the measured temperatures of low-temperature and low-fl ow hydrothermal features greatly. It is a challenge to measure the temperature of these hydrothermal features accurately.
Equipment and costs. Thermometers, digital temperature probes, and handheld TIR sensors may be purchased for tens to hundreds of dollars from a variety of vendors. Alcohol-bulb thermometers are preferable to mercury-bulb thermometers due the environmental consequences of a broken thermometer. Both thermocouple and thermistor digital temperature probes are available. Most vendors sell a variety of probes with different lengths, sheathing, and versatility. Handheld TIR sensors range from $100 and up, depending on factors such as the instrument’s precision, ability to set the emissivity, laser-pointing options, and area of ground measured.
Limitations. Even though it is relatively easy for field personnel to take single-point temperatures, these temperatures may not describe the hydrothermal feature or area adequately. For example, personnel may not know the relationship of a thermal spring’s outflow to the vent temperature of hydrothermal spring. Also, single-point temperatures do not quantify changes in the total heat of the hydrothermal feature or system. Single-point temperature measurements in wells may be useful, but do not quantify the temperature change with depth in the well (the geothermal gradient).
Logistics, repeatability of measurements and quality-control are additional limitations of using level-one temperature instruments. It may be difficult to revisit remote or dangerous hydrothermal features for repeat-temperature measurements. Also, it may be difficult to repeat measurements of temperatures at the same location due to changes in personnel or inadequate documentation of location. Temperature-measuring instruments require calibration to confirm the accuracy of temperature measurements. Calibration of temperature equipment involves maintenance and requires an investment of personnel and time.
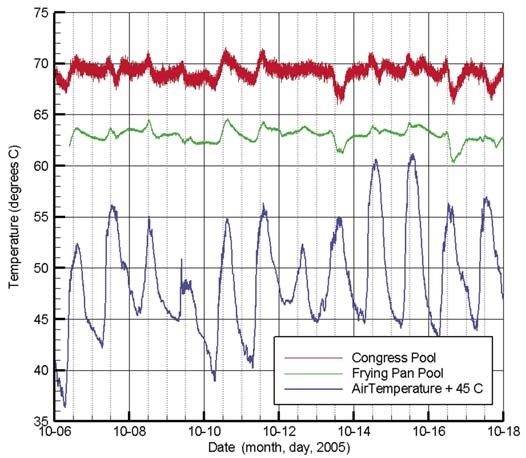
For example, at noon on 16 October, the air temperature is at maximum whereas the temperatures of the hydrothermal features are at a minimum. Note that the air temperature has been increased by 45 °C for the purpose of graphical clarity.
Graphing time-temperature relationships allows the comparison of thermal feature temperatures to temporal events. Time variant parameters include air temperature, precipitation, seismicity, or human-related activities. Graphing temperature with respect to any of these variables helps to show the natural variability within the hydrothermal feature or system.
Visualization and GIS programs allow the production of maps or three-dimensional graphics that assist the spatial analysis of temperatures. The spatial distribution of temperatures allows personnel to define and map hot and cold hydrothermal areas or features. If several maps of temperatures exist, then personnel can clearly identify the changing temperature of hydrothermal features or areas as a function of time.
Level Two: Temperature Data Loggers
Methods. A temperature data logger increases the amount of temperature data gathered at a thermal feature. Temperature data loggers are electronic devices that automatically record temperatures at user-defined temporal intervals. Similar to handheld digital probes, temperature loggers have variable precisions, accuracies, and probe construction.

Methods. A temperature data logger increases the amount of temperature data gathered at a thermal feature. Temperature data loggers are electronic devices that automatically record temperatures at user-defined temporal intervals. Similar to handheld digital probes, temperature loggers have variable precisions, accuracies, and probe construction.
The placement of temperature data loggers is similar to the placement of handheld temperature probes. A good site for placing a temperature data logger includes (1) safe access, and (2) a representative temperature of hydrothermal feature or area (Fig. 10). When placing a temperature data logger, field personnel must protect the electronic device from low or high pH, high temperatures, water, animals, and vandalism.
It is important to select appropriate temperature precision and sampling intervals for temperature data loggers. The monitoring program’s study design determines the required temperature precision and sampling interval (Fig. 9). For example, collecting a temperature every five seconds in a stable hydrothermal feature generates more data than is necessary. Another factor affecting temperature precision and sampling intervals is the data logger’s memory. High-precision and frequency of sampling requires more field visits to download temperature data.
Field personnel use a variety of techniques to download temperature data loggers. Some data loggers require a connection to a laptop computer. Other data loggers utilize a small shuttle to download field data. After download, field personnel connect the shuttle to a computer for final download. Radio-telemetered temperature data loggers are available, but require a high level of maintenance.
Calibration of temperature data loggers is an important component of the equipment’s use. An easy calibration check is the ice-point calibration (see discussion in Vital Sign 3). Most manufacturers of temperature data loggers will conduct calibrations for an additional fee.
For logging temperatures in wells, winch systems allows field personnel to lower data loggers into a well and record temperature as a function of depth. Such temperature-depth data defines the geothermal gradient and water flow characteristics for the well (Ziagos and Blackwell, 1981; Ge, 1998).
Timing and frequency. Temperature data loggers generate a more complete data set for the hydrothermal feature than level one temperature data. The frequency of automatic measurements depends upon the thermal characteristics needed for measurement. For example, to capture geyser eruptions, intervals of five seconds to one minute may be necessary. For a large hot spring, time intervals of one hour to one day may be sufficient. Temperature data loggers may remain in the field for up to one year if the battery is viable. The data logger, however, will require multiple visits per year to download data. The logging interval and logger memory determines the number of visits per year.
Equipment and costs. The cost of temperature data loggers varies with the precision of probes, amount of memory, and download method. Temperature data loggers range from one hundred dollars to over a thousand dollars. Most manufacturers supply different probe lengths, probe configurations (surface probes, immersion probes, etc.). Basic winch-type temperature loggers for the measurement of temperatures in wells are available for hundreds to thousands of dollars.
Limitations. Downloading temperature logger data in the field is a major limitation. Issues include: (1) safe access to temperature loggers for manual download; (2) time and effort for downloading multiple networks of temperature loggers; (3) maintenance of the data loggers (battery replacement, probe replacement, and calibration); and (4) personnel time. Setting parameters for logging temperature data, download of temperature data, analysis of temperatures, and conversion of temperature data to different formats requires specialized software.
Interpretation. The interpretation of data from temperature loggers follows similar techniques described under the level-one discussion. Level-two temperature data is more complete than level-one temperature data. Personnel can correlate temperature data with temperature of other hydrothermal features (Fig. 9), air temperature, barometric pressure, seismicity, and anthropogenic activities more precisely than with level-one temperature data.
The interpretation of temperature-depth data is beyond the scope of this chapter. However, many references discuss the importance and interpretation of downhole temperature measurements (Ziagos and Blackwell, 1981; Ge, 1998).
Level Three: Forward-Looking Infrared Detectors, Aerial Photography or Satellite Imagery
Methods. Humans view the world by gathering visible information with their eyes and sensing the warmth of the sun or other heat sources. Our eyes are our visible sensors that see various combinations of reflected blue, green, and red light from objects. Our hands can sense or feel the heat emitted by hot, glowing objects such as burning wood.
Both visible light (0.4–0.75 microns) and infrared energy (~1 micron to 1 cm) have spectrums of energies (Table 3). Instruments can detect or sense reflected visible energy (less than 1 micron wavelength) or radiated thermal-infrared energy (3.0–14.0 microns). Reflected infrared energy (~1−2.5 microns) occupies the portion of the electromagnetic spectrum between visible energy that our eye can see and the heat energy, or thermal-infrared energy that humans can feel. Plants strongly reflect this near-infrared energy and photographers can take advantage of this effect to create dramatic photographs.
There are different types of thermal-infrared sensors. Each thermal-infrared sensor has its advantages, disadvantages, and mapping applications such as monitoring active volcanoes, mapping volcanic eruptions, monitoring fires, or monitoring hydrothermal areas (Table 4). The size of the thermal pixels or picture elements (spatial resolution), width of the thermal band (spectral resolution), type of sensor, and instrument platform determine the applications. Thermal-infrared detectors can be handheld forward looking infrared radiometers (FLIR) or mounted on an aircraft or satellite. Aircraft may carry broad-band or narrow-band thermalinfrared sensors. Broad-band thermal-infrared sensors generally operate at 8–12 microns or 3–5 microns. Satellites with thermal-infrared sensors include: the advanced spaceborne thermal emission and reflection radiometer (ASTER), the moderate resolution imaging spectrometer (MODIS), LANDSAT and the advanced very-high resolution radiometer (AVHRR). The MODIS/ASTER simulator, or MASTER (Hook et al. 2001) is an airborne thermal-infrared sensor that acquires thermal-infrared energy in 25 discrete bands at moderate spatial resolution.
Some uses of thermal-infrared sensors for monitoring temperature and heat from volcanoes, mapping hydrothermal minerals and estimating radiative heat flux can be found in the literature. Pergola et al. (2004) used the low-spatial resolution but high-repeat AVHRR satellite imagery to detect thermal areas associated with active volcanism. Pieri and Abrams (2004) used ASTER satellite imagery to monitor active volcanoes. Carter et al. (2006) and Vaughan et al. (2005; 2006) integrated ground (FLIR), airborne (MASTER) and satellite (ASTER) sensors for mapping volcanic activity and thermal areas. Kahle (1987) used NASA’s experimental thermal-infrared multispectral scanner (TIMS) for mapping mineral alteration, as well as calculating surface emittance, temperature, and thermal inertia. Watson et al. (2008) used a LANDSAT satellite image to calculate a park-wide, radiative heat flux for Yellowstone. Using an airborne FLIR camera, Neale et al. (2008) acquired nighttime high-spatial resolution (~1 m) thermal-infrared imagery and generated a calibrated temperature map (Fig. 11) for Yellowstone’s Upper Geyser Basin. Thus, ground, airborne, and satellite thermal-infrared sensors can map and monitor the hydrothermal activity within individual basins or over a broad region.
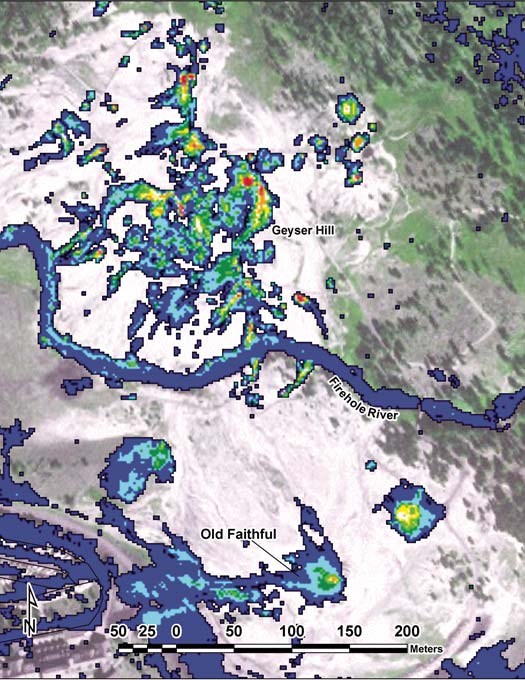
Darkest blue represents low temperatures between 5 and 10 °C, and red shows high temperatures between 10 and 60 °C. Thermal-infrared imagery courtesy of Christopher Neale and graduate students at Utah State University and Bayani Cardenas at UT.
Thermal-infrared imagery is available from a variety of commercial, government, and other sources. Low-spatial resolution satellite imagery (thermal-infrared) is available from a variety of vendors. Under contract or collaborative agreements, commercial companies, universities, and government agencies will fly over hydrothermal areas and deliver oriented, georectified and GIS-ready, thermal-infrared mosaics of high-spatial resolution imagery. The cost of software for processing, display, and analysis of images varies. Image processing, data acquisition, flight time, and personnel are the major costs in level three hydrothermal monitoring efforts.
Limitations. Equipment costs, highly skilled personnel, and time for surveys limit the frequency of temperature calibrated, high-precision, nighttime thermal-infrared imagery of active hydrothermal areas. In addition to specialized software, trained personnel are necessary to acquire and process high-accuracy, thermal-infrared imagery.
Several limitations affect the use of nighttime thermal-infrared imagery for mapping the spatial extent of active hydrothermal areas. These limitations include weather, adequate ground control points, equipment costs, image acquisition costs, image processing costs, and skilled personnel. Ground control points or LiDAR images are necessary for rectifying thermal-infrared images to known locations on Earth’s surface. In undeveloped areas, it is challenging to accurately georectify daytime, visible imagery. Nighttime, thermal-infrared imagery adds to the complexity. Highly trained personnel also must ensure that image acquisition, geometric corrections, and processed imagery meets the goals of the thermal-infrared survey of active hydrothermal systems. Specialized image processing software is necessary for the display and accurate rectification of individual airborne images into a mosaic and analysis of airborne imagery. A temperature calibrated, high-spatial resolution, georectified mosaic using thermal-infrared airborne imagery is a very time-intensive and expensive process for large areas.
Interpretation. Nighttime thermal-infrared imagery of hydrothermal areas has many uses. Scientists can construct a baseline map of temperature; efficiently and safely estimate temperatures over large area; calculate the heat flux of hydrothermal sub-basins; and document changes within an entire hydrothermal system. Earth scientists also may use the thermal-infrared imagery to construct maps of fracture networks affecting hydrothermal systems (see Case Study II). Additionally, thermal inertia maps may show how surficial geology affects a hydrothermal system if nighttime and daytime thermal-infrared imagery is acquired. Because earth materials (volcanic rocks, sedimentary rocks, sediments such as clays or sands, etc.) gain and lose heat at different rates, an interpreted new map of geology may be generated from a thermal image and integrated with existing geologic maps or any visible imagery.
Vital Sign 4: Thermal Water Discharge
A key parameter for monitoring geothermal systems is the amount of thermal water discharged (volume of water per unit time). By measuring and monitoring the thermal water discharged by both hydrothermal features and hydrothermal systems, personnel can document natural variability and anthropogenic disturbances affecting a hydrothermal system. By measuring the thermal water flowing from the hydrothermal system, we can estimate the heat from the hydrothermal system using Equation 1:
where ΔQ is the change in heat, c is the specific heat of the material, m is the mass, and ΔT is the change in temperature. For example, the amount of heat lost from 1000 g of water (one liter) cooling from 90 °C to 20 °C is 293,020 J or 10,003 calories. (The specific heat of water is 4.186 J/gram °C. From equation one, the change in heat = (4.186 J/gram °C) × (1000 g) × (70 °C) = 293,020 J or 10,003 calories.)
Techniques for measuring the volumetric discharge of hydrothermal waters are identical to the methods used in surficial hydrologic studies. Nolan and Shields (2000); Rantz (1982), Buchanan and Somers (1969), International Organization for Standardization, (1983), and Sauer and Meyer, (1992) provide detailed discussions of surface-water discharge measurement techniques.
Level One: Estimate Volumetric Discharge
Methods. Two simple methods exist for estimating volumetric discharge: (1) measuring cross-sectional area and surface velocity, and (2) measuring volume and time. The first method involves measuring the cross section area of a channel and the velocity of the water flowing through the channel. Equation 2 relates volumetric discharge (Q) to the cross-sectional area (A) and velocity of the stream (V):
Qvolumetric = (Across section)(Vstream)
Field personnel usually can measure the cross section area of the channel directly with a tape measure. It is important that a rectangular cross sectional area exists over a sufficient length of stream. The time required for an object to travel a known distance in the stream can be used to calculate the stream’s velocity. Speed-sensing devices that use radar may help with velocity estimates. Field personnel should be aware that the surface velocity of the stream may not be equal to the stream velocity at depth.
For the second volumetric technique, field personnel measure water flow with a container of known volume. At low-flows cascading over a barrier, field personnel place a container under a single stream of water, allow the container to fill with water and note the time required to fill the container. Equation 3 relates volumetric discharge (Q) to the volume of water (v) and time (t):
Qvolumetric = (vwater)(t)
Multiplying the container’s volume by the time required to fill the container yields volumetric discharge. As with any measurement, field personnel should take multiple readings and average the results for an estimated discharge.
Field personnel working with hot, caustic, acidic, and toxic flowing thermal water should ensure their safety and the safety of their coworkers. Thermal burns from splashing or immersion in water may occur. Chemical burns caused by acidic or caustic waters also may occur. Some thermal waters contain toxic elements such as mercury and arsenic. It is important for the field team to have conducted a literature review of the chemistry and site conditions of the hydrothermal feature before taking flow measurements.
Timing and frequency. At a minimum, field personnel should measure thermal water discharge at high-flow and low-flow conditions. Maximum flow usually occurs at the time of spring runoff. Minimum flows generally occur in late fall or winter. Personnel may use monthly flow estimates to calculate an annual volumetric discharge and associated annual heat flux.
Equipment and costs. Tape measures are readily available and inexpensive. Field personnel may use any container once it is calibrated and marked. A simple volumetric calibration involves filling the container with a known volume of water. The primary cost is personnel time.
Limitations. These level-one volumetric techniques are approximations of volumetric discharge that may be in error by 25% or more. The container technique can be more precise and accurate for low flows. At high flows or when the bucket cannot be easily filled, however, this technique can produce significant errors. Accurate volumetric discharges require expensive and time-consuming techniques such as wading measurements, or the installation of a flume or weir.
Interpretation. Graphs of water flow as a function of time are the simplest way to view hydrologic discharge. These graphs allow personnel to analyze the temporal variations of discharge at a single location. Also, these volumetric graphs allow a comparison of discharge for different hydrothermal features and areas. Graphing other variables allows the comparison of hydrothermal discharge to precipitation, seismicity, or anthropogenic activities.
Level Two: Wading Measurements, Weirs or Flumes
Methods. Wading measurements are a standard way to determine volumetric discharge. This technique divides a stream into a series of small cross sections. Field personnel measure stream velocities in each of the small, cross-sectional areas with an instream velocity meter. Essentially, this technique sums the volumetric discharge from each small cross section, thus yielding a total volumetric discharge for the stream. Nolan and Shields (2000), Rantz (1982), and International Organization for Standardization (1983) fully describe this technique.
Although the technique is conceptually simple, there are many factors that must be considered to make a good discharge measurement. An important consideration for a wading measurement is the location of the cross section. The stream’s channel should be straight and as rectangular as possible at the cross section. Taking measurements at bends in the stream channel is difficult and should be avoided. Ideally, the stream bed will be clear of boulders and vegetation, and relatively smooth. When these conditions are met, there is usually laminar (smooth, not turbulent) flow in the channel, which yields more accurate measurements. If repeat measurements are planned at a cross section it is helpful to place markers on the section so the same section can be reoccupied in later measurements. Nolan and Shields (2000) provide an in-depth discussion of wading measurements, cross section selection, and sources of measurement error.
Flumes and weirs are measurement structures that are placed in streams. In these calibrated structures, the height of water flowing directly over the weir or through the flume relates to discharge. While there are many types, each flume and weir has its advantages and disadvantages (Buchanan and Somers, 1969). Key parameters in the design and placement of weirs and flumes include maximum and minimum volumetric discharges, stream gradient, and stream bed characteristics. Field personnel can read the height of water in the flume or weir and look up a volumetric discharge in a rating table. Additionally, a pressure transducer may be placed to automatically measure water heights at specific time intervals. Consultation with skilled personnel trained in weir and flume placement and their installation is highly recommended.
Field personnel should record all data in a waterproof field notebook with an all-weather pen, then enter the data into a spreadsheet or database. Recorded data should include: date, time, location of measurement, datum, name of field personnel, type of volumetric measurement, parameters measured for volumetric discharge, and any observations. Cross-section and velocity data should be archived and saved. Location and photographic documentation of the measurement site is important for consistency and comparison with future discharge measurements.
Timing and frequency. Timing and frequency considerations for level-two measurements are similar to the considerations listed for level-one hydrologic measurements. At minimum, field personnel should measure hydrologic discharge at high-flow and low-flow conditions. Maximum flow usually occurs at the time of spring runoff. Minimum flows generally occur in late fall or winter. Personnel may use monthly flow estimates to calculate a yearly volumetric discharge and associated heat flux.
Equipment and costs. Wading measurement equipment includes wading rods and velocity sensors. This equipment is available from a variety of vendors. Wading rods measure the depth of water for each cross section. Velocity sensors mount directly on the wading rod and measure the water velocity. Some sensors are integrated with digital data loggers and displays, while mechanical sensors require headphones to listen to an audio signal. Wading rods generally cost a few hundred dollars. Velocity sensors and associated displays can range from one thousand to many thousands of dollars.
The cost of weirs and flumes varies greatly. Using a standard design, field personnel may construct weirs from notches cut into sheet metal. In low-flow conditions, personnel can place the weirs and flumes directly into the stream. For high-flow conditions, field personnel and hydrologic experts need to engineer and construct concrete supports. The advice of a hydrologic expert is invaluable in selecting a weir or flume for a specific hydrologic setting.
Limitations. Location, stream characteristics, and total volumetric discharge affect the quality of wading measurements. Imprecise volumetric discharge results from a non- rectangular cross section, high bed roughness or high stream bank roughness. Very low-flow and high-flow conditions are difficult to measure with wading techniques. Precise and accurate wading measurements require highly skilled field personnel. Wading measurements done by experienced personnel have errors of ~3–10%.
Interpretation. Wading, flume, and weir measurements are more precise measurements of discharge than bucket tests or the surface velocity cross-sectional area method. The interpretation of level-two measurements is similar to level-one measurements. Level-two discharge measurements and fluid temperature measurements also allow an estimate of heat transfer by water using Equation 1:
ΔQ = cmΔT
Graphs of water flow as a function of time are the simplest way to view hydrologic discharge. These graphs allow personnel to analyze the temporal variations of discharge at a single location. With data from a pressure transducer, the frequently collected discharge data allows very precise temporal correlations among different hydrothermal features and hydrothermal areas, as well as with precipitation, seismicity, or anthropogenic activities.
Level Three: Real-Time Gauging Stations
Methods. Stream-flow gauging stations provide a continuous record of discharge. Site selection for a gauging station is similar to selection of a cross section for a wading measurement. Additional requirements are the assessment of stream bank and bed stability for the location of instrumentation and for long-term stability of the site. At a gauging station, the stage or elevation of the stream surface is measured with a pressure sensor in the
river. Stream discharge is computed by correlating the stage of the stream with stream flow measured using wading measurements (Buchanan and Somers, 1969). The resulting correlation (a rating curve) relates river stage to stream discharge. Stream gauges have data loggers to record the stage measurement. In some cases, telemetry allows near real-time preliminary volumetric discharges to be computed. These data can be displayed on base station computers or on the Internet. Users can set the interval for data acquisition and subsequent transmission. Field personnel should conduct wading measurements every one to two months to confirm the validity of the rating curve and discharge calculations (Buchanan and Somers, 1969). The stage datum at a gauging station needs to be periodically remeasured so the conversion of stage to discharge can be recalibrated. This is especially important if active erosion or deposition is taking place at the gauging station site.
Gauging stations can collect and transmit other real-time data such as water temperature, air temperature, specific conductance, and rainfall. The development of new chemical probes holds promise for the real-time measurement of selected ions such as chloride (Fig. 12).

Equipment and costs. The cost of a real-time gauging station depends upon the type of pressure sensor, data, and transmission equipment, and on personnel costs associated with the physical measurements and maintenance of the gauge. Pressure sensors can vary from pressure transducers that cost hundreds of dollars to bubbler systems that cost thousands of dollars. The cost of electronic data transmission equipment depends upon the method of data transmission. Short-distance, local-area-network transmission and reception equipment may be obtained for less than $10,000. Satellite-telemetered equipment is more expensive, in the tens of thousands of dollars.
Data acquisition, quality assurance, and archiving data require specialized equipment and software. Due to the high volume of real-time data, the storage, processing, and archive of the gauge data is a significant cost. Current U.S. Geological Survey costs for installation of a real-time satellite gauging station are about $40,000, with an annual cost of $16,000 to $20,000 for operation and maintenance.
Limitations. Key limitations with real-time gauging stations are the expertise needed to install, maintain, process, and archive data. Knowledge of stream flow, hydrologic measurements, telemetry of data, database management, systems administration, and secure archiving of data are necessary.
Interpretation. Graphing time-discharge relationships allows the comparison of thermal feature discharge to any temporal event. The near-continuous discharge measurements and precise temporal correlations allow the effects of precipitation, seismicity or anthropogenic activities to be correlated very precisely with thermal discharge. The real-time data also allows field personnel and managers to respond in a timely fashion to changes in the hydrothermal flow.
The locations, temperatures and flow rates of thermal sites (Vital Signs 1 through 4) provide valuable data that should be supplemented with chemical analyses of thermal and local nonthermal waters. Constituents dissolved in hydrothermal waters can include common anions and cations, trace elements, organic compounds, isotopes, and radio nuclides. Hydrothermal flow systems are complex (Henley and Ellis, 1983) and often water chemistry analyses are the best way to assess subsurface flow systems.
Kharaka et al. (1991), summarizing their work and the work of Hem (1985) and Ellis and Mahon (1977), note that chemical data, among other uses, can help determine:
• baseline chemistry of hydrothermal systems to determine the natural range of variation of individual features and an overall system;
• subsurface temperatures;
• subsurface mixing of different fluids (for example, deep hydrothermal and local cold water);
• water-rock interaction parameters that might be occurring at depth;
• water flow paths;
• interactions between thermal and non-thermal groundwaters;
• the origin of chemicals in hydrothermal waters;
• hydraulic connections among widely spaced thermal sites;
• possible contributions of magmatic water to hydrothermal systems; and
• the age of hydrothermal systems, through dating waters or hydrothermal deposits.
These are all valid goals for monitoring, as both local and distant stressors can influence the resulting chemistry of a thermal site. Individual land managers will need to assess which of these uses of chemical data are most appropriate for their areas.
Level One: Electrical Conductivity, pH
Methods. Electrical conductivity and pH are two characteristics of geothermal fluids that field personnel can measure easily. Electrical conductivity is a measure of the ionized chemicals in water. Generally, high amounts of dissolved minerals and salt concentration in water yield high electrical conductivities. Measurements of electrical conductivity can provide estimates of the hydrothermal fluid’s purity and contribute to understanding its flowpath.
A measure of the hydrothermal fluid’s acidity or alkalinity is its pH; a pH of 7 is neutral, less than 7 is acidic, and above 7 is basic. Changes in the pH may indicate changes in the flow path of the geothermal fluid, changes in the temperature of the geothermal fluid, loss of CO2, or anthropogenic activities.
To measure conductivity, field personnel immerse a calibrated conductivity probe in water. Prior to making a measurement of electrical conductivity, the field team should calibrate the meter and probe with solutions of known conductivity and according to manufacturer’s guidelines. When placed in water, the calibrated probe requires time for the reading to stabilize, or equilibrate, to the fluid being measured. After equilibration, the meter displays the fluid’s electrical conductivity.
A pH meter or pH paper can be used for estimating the pH of a geothermal fluid. Personnel dip the pH test-strip in the geothermal fluid, watch for color change, compare color changes in the test strip with a color scale, and read the result. pH determined by the use of paper may not be highly accurate. A pH meter’s use is similar to a conductivity meter. Prior to making a measurement of pH, field personnel should calibrate the probe with solutions of known pH and according to manufacturer’s guidelines. When placed in water, the calibrated probe requires time for the reading to stabilize, or equilibrate, to the fluid being measured. After equilibration, the meter displays the fluid’s pH. It is critical that field personnel calibrate the meter and probe according to manufacturer’s guidelines.
Field teams should record all data in a waterproof field notebook with waterproof pens. Personnel should then enter the field data into a spreadsheet or digital database. Recorded data should include: date, time, coordinates of measurement, name of field personnel, type of pH and conductivity equipment, pH, fluctuations of pH, temperature of pH measurement, calibration information concerning the pH meter, conductivity, fluctuations of conductivity, temperature of conductivity measurement, calibration information concerning the electrical conductivity meter, and any observations about the fluid being measured. Location and photographic documentation of the measurements sites are important for consistency and comparison with future measurements.
Timing and frequency. Two key factors affecting timing and frequency are study design and accessibility. Once electrical conductivity and pH baselines are established, the frequency of measurements can decrease. Initially, field teams should take electrical and pH measurements frequently to define any daily or seasonal variations. These simple measuring techniques may not monitor remote hydrothermal features adequately or allow the determination of a baseline. Adequate baseline chemical data depends upon the questions asked and answers needed. The questions and expected baseline data are part of the geothermal monitoring study design. For initial reconnaissance, a remote backcountry hydrothermal feature unaffected by human activities may require annual monitoring. However, a hydrothermal feature affected by human activities may require monthly or weekly monitoring of pH and electrical conductivity. As with any monitoring effort, careful compilation and documentation of results from previous studies is critical.
Equipment and costs. Electrical conductivity meters are available from a variety of vendors. The cost is generally a few hundred dollars, with calibration standards costing tens of dollars. Replacement probes are available for about $100.
A variety of vendors sell pH paper. Range of pH paper sensitivity (0–14, 0–6, or 7–14) and type of color change (single indicator or multiple colors) vary between manufacturers. The cost is generally tens of dollars for ~20 pH paper strips.
Numerous vendors sell pH meters. The cost is generally a few hundred dollars, with calibration standards costing tens of dollars. Replacement pH probes are available for about $100. Vendors also sell combination electrical conductivity and pH probes.
Limitations. The maintenance and calibration of field pH and electrical conductivity meters requires effort. Expiration dates of calibration solutions, care of probes and meters, and maintenance and calibration all require time and documentation. Meters can fail in the field.
High-temperature geothermal fluids present challenges for precise measurement of conductivity and pH. Some conductivity and pH meters are not capable of taking accurate readings at higher temperatures (generally greater than 80 °C).
Visual comparisons of color change on pH test strips can be a challenge. Lighting conditions (full sun, part-shade, cloudy day, etc.) and the color perception of an observer affect the visual comparison of color change and the accuracy of the perceived pH.
Similar to taking temperature or hydrologic measurements, field teams must consider personal safety when taking electrical conductivity or pH measurements near hydrothermal features. Personnel may incur thermal burns from splashing or immersion in thermal water. Also, field personnel may sustain chemical burns from acidic or caustic thermal waters. Some thermal waters contain toxic substances such as mercury, arsenic, and other elements.
Interpretation. Graphs of water chemistry, pH, and electrical conductivity as a function of time are the simplest way to view the data. These graphs allow personnel to analyze the temporal variations of water chemistry, pH, and electrical conductivity at a single location. Also, these graphs allow a comparison of data between different hydrothermal features and areas. Graphing other variables allows the comparison of these data with temperature, precipitation, seismicity, or anthropogenic activities such as pumping wells. In addition, GIS maps of these variables may show important spatial and temporal trends in the data.
Methods. Levels two and three of chemical studies are separated more by the frequency of sampling than by the distinction of what elements or compounds are analyzed. Level-two studies involve fewer samples, and are more directed at monitoring surface and subsurface conditions in the vicinity of hydrothermal features. Level-three studies are larger in scope, more involved, and can extend beyond monitoring local features to providing information on the entire flow path of fluids, from recharge to discharge. Level-two studies are more typical of monitoring programs, while level-three studies provide monitoring data that comes closer to research programs.
To deal with the complexity of chemical programs, it highly advisable to work with specialists in hydrothermal fluid chemistry to assure that sampling protocols are followed exactly, that chain of custody and shipping issues are handled properly, and that a reliable lab is chosen to do the analyses. Experts can also help with interpretation of complex and large data sets.
For monitoring data to be considered reliable, chemical results must be both accurate (representative of what really exists in the field) and precise (measuring what is really in the sample with results that are able to be reproduced). Accuracy can be assured by selecting appropriate sample sites and collecting samples properly. Precision can be assessed by providing labs with duplicate blind samples, and evaluating how close the analytic data are. It may also help to check one lab against another by sending duplicate samples to multiple labs. It is also critical that analyses be performed by U.S. Environmental Protection Agency (EPA)–certified labs. While other labs may provide chemical analyses for lower costs, EPA certifi cation provides an additional level of assurance.
Timing and frequency. The timing and frequency of chemical analyses at levels two and three depends on the questions being asked. Once baselines are established, the frequency of measurements can decrease. In areas where there is little stressor influence on hydrothermal systems, sampling once every few years may be adequate. In areas where there is influence from stressors, sampling may need to occur on annual or monthly cycles. It can be useful, in dynamic areas, to sample weekly to determine the natural range of variation of individual features and an overall system (Fournier et al., 2002).
Equipment and costs. Chemical analyses of hydrothermal fluids have detailed field sampling, sample handling, and sample analysis protocols, which are different depending on the specific elements or compounds being studied. Sampling is much more than going to the field with a bucket and bringing water back to a lab. For example, sampling for multiple chemical species typically requires that some of the fluids be filtered before they are placed in bottle, that some of the fluids not be filtered, that some of the sample be collected in glass bottles, that some of the sample be collected in plastic bottles, that some of the samples be acidified in the field for preservation of chemical species, and that triple-rinsed bottles be prepared well and have the right kinds of lids.
Nicholson (1993) provides an extensive list of considerations for preparation for field work, and Mazor (1991) discusses field sampling requirements for isotopic analyses (even noting that some samples should not be exposed to sunlight). Reference to both Nicholson (1993) and Mazor (1991) will provide broad contexts for considering options and requirements for field sampling programs.
Chemical analyses can range from less than $100 per sample for some elements to more than $500 per sample for isotopes. Therefore, the distinction between levels two and three is determined by the number of individual samples and the number of elements or compounds that need to be analyzed for in each sample. Total costs for studies can range from several hundred dollars for simple studies of a few samples analyzed for major elements only, to many tens of thousands of dollars for detailed, repeat studies including analysis of large suites of samples for many elements, compounds and isotopes.
There also are costs that will be incurred as personnel are appropriately trained to collect samples. If local staff are not trained, then consultants will have to be hired to do the field sampling; this can run from hundreds to more than a thousand dollars a day, depending on the sophistication required for the sampling.
Table 5 indicates some of the more common chemical constituents of hydrothermal fluids that may be analyzed and interpreted as part of a monitoring program.
Limitations. One of the biggest requirements in hydrothermal fluid sampling programs is absolute certainty that the correct samples have been collected from the correct sites in the proper way. What is correct depends on the monitoring questions that are being asked. Sampling to provide data about subsurface temperatures is different from sampling to provide data about what rocks the hydrothermal waters have chemically interacted with at depth. Picking appropriate sites is also important. High-flow springs may provide better chemical characterization of subsurface conditions than low-flow springs (but low-flow springs may be better than no data). Improper sample collection techniques or improper shipping may invalidate chemical results.
Similar to taking temperature or hydrologic measurements, field teams must consider personal safety when taking chemical samples from hydrothermal features. Personnel may incur thermal burns from splashing or immersion in thermal water. Also, field personnel may sustain chemical burns from acidic or caustic thermal waters. Acids used to stabilize samples can also be hazardous. Some thermal waters contain toxic substances such as mercury, arsenic, and other elements.
Labs may report analytic results using different units for concentrations. All appropriate unit conversions must be made before results are interpreted. Mixing units can lead to major errors in the interpretation of results.
When interpreting chemical results, it is critical to be aware of analytic uncertainties and detection limits. Although a particular analytic result may suggest a potential interpretation, inclusion of the uncertainty associated with the analytical technique clarifies the confidence of the interpretation. Analytic results for solutes that are close to the lower limit of detection may not be as reliable as results that are well above the limit.
Interpretation. Major and trace element chemistry of hydrothermal fluids can provide data about subsurface conditions and water flow paths. These data are useful in monitoring programs, as they allow insight into deeper geologic processes, and may indicate system changes that are not visible from the surface.
One key question of subsurface conditions is the temperature of hot water at depth. Where direct measurement of deep temperatures, through measurements in wells, is not possible, water temperature at depth is determined through interpretation of water chemistry. The concept of geothermometry is simple: some ratios of elements, gases, or isotopes will become fixed at a high temperature, and the ratio will be maintained as the waters cool. Chemical determination of these ratios, therefore, can be interpreted to give an estimate of the maximum temperature of a particular hydrothermal system. Nicholson (1993) describes many of these ratios and includes appropriate formulas. Some elements that can be used for geothermometry are listed in Table 5.

The lone sample point between the two fields suggests that it is a mixed water, with both thermal and non-thermal inputs (from Foley and Street, 1988).
Although gathering field samples can be done by personnel with only a small amount of training in sampling and sample handling protocols, interpretation of chemical monitoring data is best done by experts. Identifying which aspects of the chemistry are a result of the interaction of water and rocks in the subsurface, which aspects are the result of mixing warm and cold waters, which aspects may be the result of subsurface boiling, and which aspects may be changing because of input from stressors, can be complex.
The discussion above has emphasized monitoring the chemistry of hydrothermal waters. It may also be appropriate, in some cases, to study deposits from hydrothermal systems. Elements in siliceous sinter or calcareous travertine may provide clues to the ages of hydrothermal activity (Kharaka et al., 1991; Foley, 2006).
Advanced Monitoring Techniques that Measure Multiple Vital Signs
Gas emissions from hydrothermal systems and changes in seismic activity, as well as changes in Earth’s magnetic, electrical, and gravity fields, can all be monitored as vital signs. Study of these vital signs, however, typically involves professional expertise and sophisticated equipment, making them best suited to level-two or level-three monitoring, or research activities. Geophysical monitoring, for example, often involves expensive equipment, logistic support issues, and, for some techniques, field deployments that can last for months or longer.
Hydrothermal Gases
Although many hydrothermal gases and their isotopes can be studied for research, monitoring programs for H2S and CO2 can help identify sites of environmental concern, as well as provide fundamental information about a local system. Both H2S and CO2 can be toxic (National Institute for Occupational Safety and Health, 1981), as they are denser than air and can settle in low areas. It may be important to pay attention to place names. In Yellowstone National Park, places named Death Gulch and Poison Spring have been sites of recorded wildlife deaths from gas poisoning.
High concentrations of H2S are typically found in volcano-related hydrothermal systems where there may be hightemperature systems at depth, or in hydrothermal systems with sulfur deposits or high organic contents in subsurface rocks (Nicholson, 1993). H2S can also be concentrated in areas with subsurface boiling, such as fumaroles and mud pots. In systems where H2S is a concern, gas sampling should be done under a variety of weather conditions to establish typical background concentrations. Portable H2S meters cost more than $500. The data must be carefully interpreted, as a wide range of concentrations may be measured, depending on wind dispersal of gases.
Other than water vapor, CO2 is typically the most abundant gas emitted from hydrothermal systems. It is found in a wide range of hydrothermal systems, including those associated with volcanoes, those where limestone rocks exist in the subsurface, and those with organic matter in subsurface rocks. In systems where CO2 is a concern, gas sampling should be done under a variety of weather conditions to establish typical background concentrations. Portable CO2 meters to do this sampling cost more than $500. The data must be carefully interpreted, as a wide range of concentrations may be experienced, depending on wind dispersal of gases.
Geophysical Methods
Hydrothermal systems can create anomalous patterns in local geophysical fields. Distinct patterns of electrical, magnetic, or gravity anomalies may indicate zones of increased permeability. Interpretation of these patterns, however, is best done by professionals with expertise in specific subdisciplines of geophysics. For example, someone familiar with electrical fields may not be qualified to interpret patterns in gravity anomalies. Another issue with geophysical methods of investigation is that there may not be unique interpretations of natural patterns. Zones that conduct electricity, for instance, may be created either by moving waters or clay-rich rocks.
Earthquakes are known to affect hydrothermal systems (Marler, 1964). Thus, the monitoring of earthquakes helps define the timing of events that may have significant effect on hydrothermal features. Hydrothermal features can also cause microseismicity (Kieffer, 1984).
Monitoring Overall Systems
Rivers that flow through or drain thermal basins can integrate all discharge from hydrothermal systems. Analyzing river waters for solute concentrations, especially Cl, is a technique that can be used to assess the overall thermal output of systems. The calculation of overall heat flux from a system or region is based on the concentration of Cl and the discharge of rivers draining the hydrothermal systems. This technique has been used in Yellowstone (Hurwitz et al., 2007; Friedman and Norton, 2007), Long Valley (Sorey, 2000) and the Cascade Range (Mariner et al., 1990).
GEOTHERMAL MONITORING STUDY DESIGN
As with any scientifi cstudy, the single most critical issue is the goal of the study. If personnel do not identify a clear goal for monitoring, then it will not be possible to select appropriate scientific techniques and analyses. A geothermal monitoring program, without a clear sense of purpose, may not meet resource protection needs. Goals of past geothermal monitoring efforts include: (1) determining if the production of water from wells was measurably affecting the flow of a hot spring (Spencer, 1986; Jarvis, 1986); (2) determining if the development of adjacent Known Geothermal Resource Areas might affect an entire hydrothermal area (Sorey, 1991); and (3) evaluating short- to long-term and seasonal variability of hydrothermal features (Cole, 1982; Ingebritsen et al., 2001).
The second critical issue in designing a geothermal monitoring plan is budget. As identified in previous sections, the cost difference between level one and three efforts may be considerable. The primary cost of most level-one studies is field personnel. The cost of level-three studies is significant because of the equipment, technical expertise, and time required for analyses of data. Although level-three studies provide the most detailed interpretations of a geothermal system, appropriately designed level-one and level-two studies can provide significant information for resource management and protection (see Case Study I).
A third critical issue in the design of geothermal monitoring plans is to identify, document and follow scientific protocols when gathering data. Simple studies can obtain critical data, if personnel follow protocols and document the methods used. If studies are not planned and implemented with care, then data might not be useful, and funds may be wasted.
Questions that must be answered are the key to designing a scientific study. The questions and clearly defined goals of a geothermal monitoring plan direct personnel toward specific scientific techniques and measurement tools. Questions that may help personnel decide what vital signs and what level of study are necessary include:
• Where are hydrothermal features located?
• How large is the hydrothermal area?
• What are baseline temperatures and fl ow levels?
• Do features change seasonally? Over a few years? Over a few decades?
• Are hydrothermal features interconnected?
• What nearby factors might infl uence specific features?
• Are human activities either at the site or on adjacent lands likely to influence the hydrothermal features?
Before starting a monitoring program, it is critical to conduct a literature review and compile information from previous studies. A large amount of data may exist about hydrothermal features and the geology of the study area. There are many sources of information that should be searched. These include scientific peer-reviewed publications, so-called gray literature (federal, state, and local government reports that have not been broadly disseminated), books of both general and local nature, internal agency reports and notes and correspondences that are in agency files, and online data, which for geothermal systems includes information from (but not limited to) the U.S. Geological Survey, the U.S. Department of Energy, the U.S. Environmental Protection Agency, the National Oceanic and Atmospheric Administration, the National Aeronautics and Space Administration, and various state geologic, land management, environmental, energy, and water agencies. County and other local agencies may also have water-related data of interest to studies of hydrothermal systems.
CASE STUDIES
Case Study I: An Inventory of All Thermal Features in Wyoming (Exclusive of Yellowstone National Park)
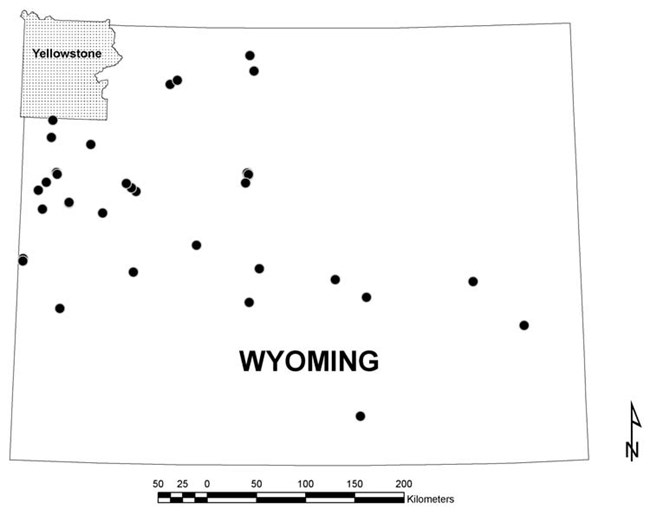
With the exception of chemical analyses, the WGS used level-one monitoring techniques. Breckenridge and Hinckley (1978) discuss the techniques used in the following excerpt from page 8 of their report:
Each spring is located on a U.S. Geological Survey topographic quadrangle by Section, Township and Range. The Section is further divided into quarter-quarter-quarters. … Temperatures were taken with both maximum and constant reading mercury thermometers. Several readings were made to determine each reported maximum spring temperature. All temperatures were taken near the surface, as close to the vents as possible. Rates of flow were visually estimated as channel crosssection area multiplied by a timed surface flow rate.…
This study is an inventory and technically not a monitoring plan, because the sites were not revisited systematically and remeasured. However, baseline inventories are very important and a key first step in developing monitoring plans. Once personnel inventory resources, they can then design an appropriate monitoring plan. This level-one and level-two inventory of Wyoming’s thermal springs formed the basis for detailed scientific studies of Wyoming’s hydrothermal resources (Buelow et al., 1986; Heasler and Hinckley, 1985; Heasler et al., 1983; Heasler, 1982; Hinckley and Heasler, 1984, 1987). Also, this study provided baseline data used to monitor and protect Wyoming’s Thermopolis Hot Springs State Park (Hinckley et al., 1982; Jarvis, 1986; Spencer, 1986).
Case Study II: Norris Geyser Basin, Yellowstone National Park
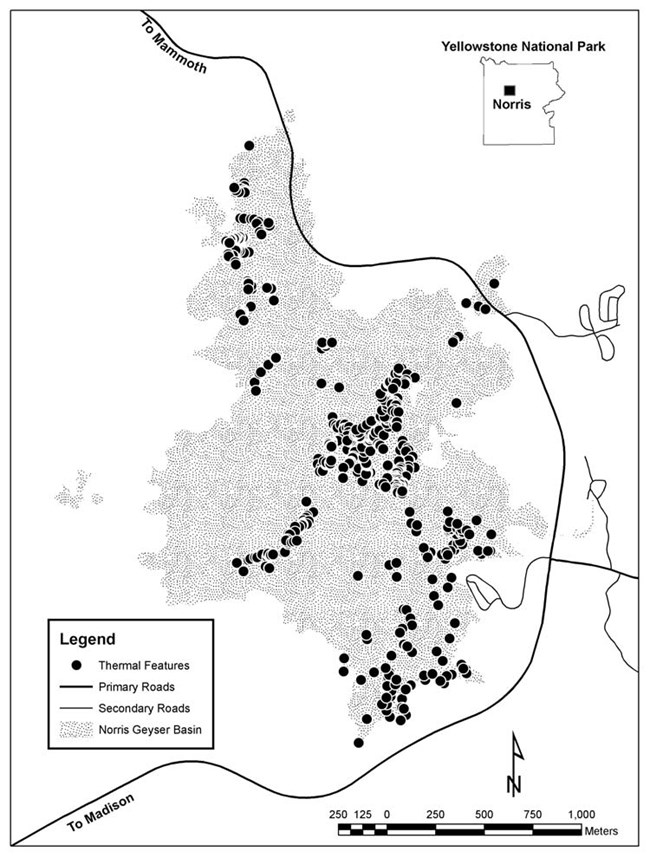
Located in northwestern Yellowstone National Park, Norris Geyser Basin is a dynamic hydrothermal area with numerous hydrothermal features and active geologic processes. Within the 5.5 km2 area of Norris Geyser Basin, field personnel mapped over 550 individual thermal features (Fig. 15). The water in these features range in pH from 1 to 8 and vary in temperature from 14 °C to 93 °C. Norris Geyser Basin includes a rare acidic geyser (Echinus). Eruptions of Steamboat Geyser at Norris are some of the largest in the world, ejecting boiling, silica-rich water up to 100 m in the air and creating a thunderous roar in its steam phase for days (Fig. 16). Norris Geyser Basin is subject to basin-wide thermal disturbances that change some geysers into fumaroles, cause hydrothermal pools to drain, and change the chemistry of features from neutral to acidic. These disturbances occur over the time scale of days to weeks and generally occur during summer and fall, but also at other times. The 1989 hydrothermal explosion of Porkchop Geyser (Fournier et al., 1991) and the 2003 closure of the Back Basin trail for three months due to high ground temperatures emphasize the dynamic nature of Norris Geyser Basin.
The monitoring plan for Norris Geyser Basin concentrates all efforts on the hydrothermal system’s heat source and its groundwater flow regime. Understanding the heat source and groundwater flow regime is critical for protecting Yellowstone’s hydrothermal resources from human activities, such as the development of energy and water resources. Level-one strategies include basic mapping, repeat photography, taking single-point temperature measurements, measuring electrical conductivity of selected hydrothermal fluids, determining pH of selected hydrothermal fluids, and estimating hydrothermal discharge with the cross-section/velocity method. Level-two strategies include GPS surveys with handheld recreational GPS receivers, deploying temperature data loggers (see Fig. 10), measuring fracture orientations in excavations for wastewater treatment facilities, and estimating hydrothermal discharge with wading measurements. Level-three strategies include the use of mapping-grade GPS receivers to perform surface hydrologic surveys, conducting on-the-ground reconnaissance of changing hydrothermal activity with a forward-looking infrared thermocam (see Figs. 7 and 8), monitoring hydrothermal discharge using a real-time stream gauge, monitoring temperature and water quality, acquiring georectified airborne imagery of hydrothermal areas at night and during the day, generating calibrated temperature maps, and calculating heat flow.

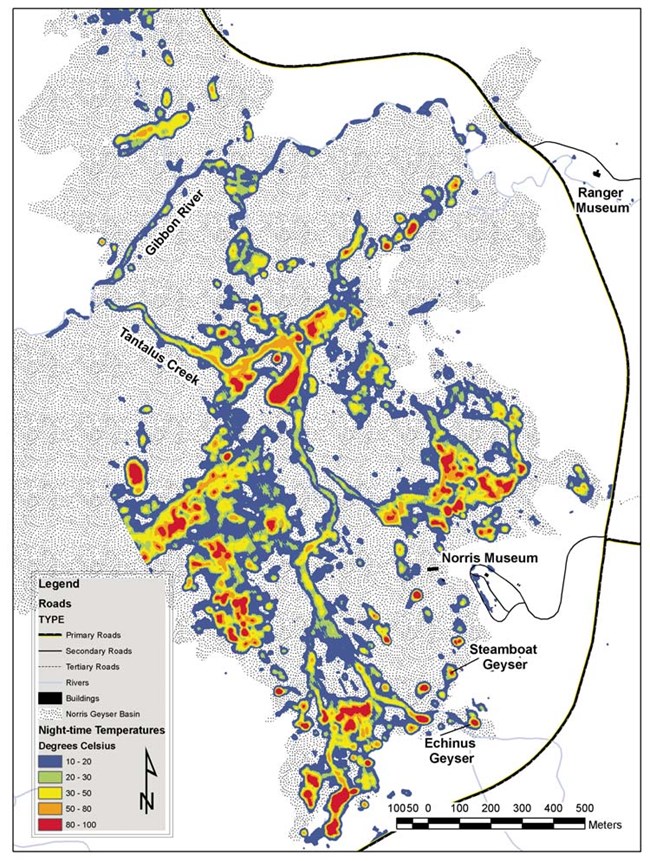
The calibration line is accurate for temperature between 10 °C and 100 °C. This nighttime, thermal-infrared mosaic clearly shows northwest- and northeast-trending alignments of hydrothermal fluids and features.
Information derived from a calibrated temperature map enhances the current understanding of the hydrothermal system and its geologic processes. Extracting the area of ground at 20 °C and examining the hydrothermal fluid flow yields a new map, showing major fractures within the underlying bedrock (Fig. 18). Scientific studies can integrate this information with other geochemical, seismic, or hydrologic data.
Highlights of a literature review clearly indicate the amount of scientific studies focused on Norris Geyser Basin. Initial studies (Gooch and Whitefield, 1888; Hague, 1911) provide the earliest geochemistry of thermal water and ideas about the Norris hydrothermal system. Subsequent scientific investigations built upon those initial investigations. Fenner (1936) recovered core and reported on early scientific boreholes, including the Carnegie II hole in Norris Geyser Basin. In addition to measuring the water chemistry of 25 thermal springs, Allen and Day (1935) established weirs near the Gibbon River, estimated discharge, and calculated a convective heat flow for Norris Geyser Basin. White (1957) improved Allen and Day’s estimates of convective heat flow. Building upon previous work, Fournier et al. (1976) calculated new estimates of convective heat flow using a chloride-inventory method. Fournier et al. (2002) also performed geochemical inventories of selected thermal springs. White et al. (1975) reported on scientific drilling during the 1960s, including the Y-9 and Y-12 drill holes in Norris Geyser Basin. Geologic mapping by Richmond and Waldrop (1975) and Christiansen (1975) provided base maps for subsequent investigations. White et al. (1988) discussed the basin’s geologic setting, chemistry of thermal waters, mineral precipitates, fractures, thermal disturbances, and geologic history. Kharaka et al. (1991) investigated the geochemical and hydrologic connection between the Corwin Springs known geothermal resource area and hydrothermal features along the Norris-Mammoth Corridor. Friedman and Norton (2000) measured chloride in various streams and calculated the chloride-flux for Yellowstone’s major streams. Ball et al. (1998a, 1998b, 2001, 2002) conducted regular geochemical surveys of thermal waters including Norris Geyser Basin. This rich history of scientific studies allows today’s scientists to use previous observations, drill logs, core from boreholes, estimates of heat flux, chemistry, fracture data, and geologic mapping to compare existing information with new data. Scientists can use all this information to develop new models about the Norris hydrothermal system.

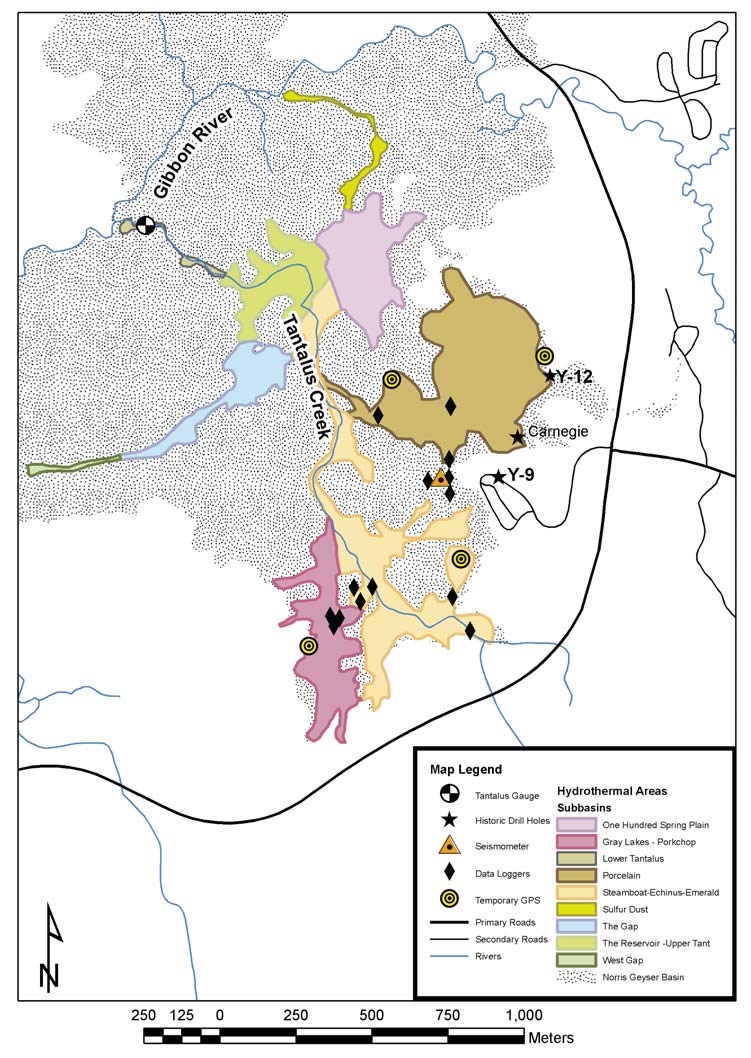
There are many reasons to monitor hydrothermal systems and develop a hydrothermal monitoring plan. Clearly identifying the goals of a hydrothermal monitoring plan focuses efforts on monitoring vital signs and employing appropriate techniques. Goals for thermal monitoring may meet legislative mandates, protect geothermal resources, provide for human safety, document anthropogenic disturbances or natural changes, provide baseline information, assess the effects of hydrothermal system on plants and animals, protect natural geologic processes, and support scientific studies.
With limited time and financial resources, personnel must assess their priorities and the important characteristics of a hydrothermal system (Table 6). Changes in water quantity, changes in the heat source, changes in rock permeability, and human activities interact with the geologic system to produce observable changes in a hydrothermal area. These observable changes are vital signs. Vital signs of hydrothermal systems include the location of hydrothermal features, spatial extent of hydrothermal areas, temperature and heat flow, thermal water discharge, and fluid chemistry. Personnel can choose from a variety of methods with different levels of expertise, equipment, and cost for measuring each hydrothermal vital sign.
Inventories, baseline data collection, monitoring efforts and scientific studies all provide information for protection of hydrothermal resources (Fig. 20). Ideally, volunteers, resource specialists, and scientists all interact as a team whose various levels of study build upon each other. First, volunteers, resource specialists, or scientists should conduct an inventory of the resource. Inventories may include mapping location, measuring temperature, estimating water discharge, and determining chemistry of individual hydrothermal features or areas. Second, resource specialists or scientists should define baseline conditions, collect spatial and temporal data, and monitor changes in the hydrothermal system. Third, scientific researchers should conduct studies of the hydrothermal system and develop an understanding of the physical processes controlling the hydrothermal system as well as system interactions. With these efforts and information, managers can develop strategies, implement management practices and effectively protect hydrothermal systems.

The paper greatly benefi ted from reviews by Jake Lowenstern, scientist-in-charge of Yellowstone Volcano Observatory; Joe Moore, Energy and Geoscience Institute, University of Utah; David Susong, U.S. Geological Survey; and Larry Martin, National Park Service. Moore also provided extensive information about geothermal systems. The authors also thank Utah State University professor Christopher Neale and graduate students at his Remote Sensing Services Laboratory (Saravanan Sivarajan and Osama Akasheh) and Bayani Cardenas at University of Texas for their thermal-infrared remote sensing efforts. The authors are also grateful to David Blackwell, Andrew Eaton, Clayton Nichols, and Marshall Reed for their helpful comments and guidance, and to Ron Keim, Katherine Luketina, and Bridgette Lynne, who provided information about monitoring in New Zealand.
REFERENCES CITED
Introduction
Barrick, K.A., 2007, Geyser decline and extinction in New Zealand—Energy development impacts and implications for environmental management: Environmental Management, v. 39, p. 783–805, doi: 10.1007/s00267-005-0195-1.
Berry, G.W., Grim, P.J., and Ikleman, J.A., 1980, Thermal Spring List for the United States: National Oceanic and Atmospheric Administration Key to Geophysical Records Documentation No. 12, 59 p., 3 plates.
Chaffee, M.A., Shanks, W.C., III, Rye, R.O., Schwartz, C.C., Adams, M.G., Carlson, R.R., Crock, J.G., Gemery-Hill, P.A., Gunther, K.A., Kester, C.L., King, H.D., and Podruzny, S.R., 2007, Applications of Trace-Element and Stable-Isotope Geochemistry to Wildlife Issues, Yellowstone National Park and Vicinity, in Morgan, L.A., ed., Integrated geoscience studies in the greater Yellowstone area—Volcanic, tectonic, and hydrothermal processes in the Yellowstone geoecosystem: U.S. Geological Survey Professional Paper 1717, 532 p.
Durand, M., 2006, Indoor air pollution caused by geothermal gases: Building and Environment, v. 41, no. 11, p. 1607–1610, doi: 10.1016/j.buildenv. 2005.06.001.
Foley, D., 2006, Dating Castle Geyser: Preliminary results and broad speculations on the geologic development of geysers and hydrothermal systems in Yellowstone National Park, Wyoming, USA: Geothermal Resources Council Transactions, v. 30, p. 413–417.
Fournier, R.O., Thompson, J.M., Cunningham, C.G., and Hutchinson, R.A., 1991, Conditions leading to a recent small hydrothermal explosion at Yellowstone National Park: Geological Society of America Bulletin, v. 103, p. 1114–1120, doi: 10.1130/0016-7606(1991)103<1114:CLTARS>2.3. CO;2.
Fournier, R.O., Christiansen, R.L., Hutchinson, R.A., and Pierce, K.L., 1994, A fi eld-trip guide to Yellowstone National Park, Wyoming, Montana and Idaho—Volcanic, hydrothermal, and glacial activity in the region: U.S. Geological Survey Bulletin 2099, 46 p.
Green, B.D., and Nix, R.G., 2006, Geothermal—The energy under our feet: National Renewable Energy Laboratory, Report NREL/TP-840-40665, 20 p.
Holdmann, G., 2007, The Chena Hot Springs 400 kW Geothermal Power Plant, Experience gained during the first year of operation: Geothermal Resources Council Transactions, http://www.geothermal.org/powerpoint07_tues.html, downloaded 18 March 2008.
Jackson, J.A., 1997, Glossary of Geology: American Geological Institute, fourth edition, 769 p.
Kennedy, B.M., and van Soest, M.C., 2006, A helium isotope perspective on the Dixie Valley, Nevada hydrothermal system: Geothermics, v. 35, p. 26–43, doi: 10.1016/j.geothermics.2005.09.004.
Marler, G.D., 1956, How old is Old Faithful Geyser?: American Journal of Science, v. 254, p. 615–622.
McKenna, J.R., and Blackwell, D.D., 2004, Numerical modeling of transient Basin and Range extensional geothermal systems: Geothermics, v. 33, p. 457–476, doi: 10.1016/j.geothermics.2003.10.001.
Moore, J.N., 2007, The hydrothermal framework of geothermal systems: Geothermal Resources Council, workshop on Geophysical Techniques in Geothermal Exploration, http://www.geothermal.org/powerpoint07_workshop.html, downloaded 17 March 2008.
Schuster, J.E., and Bloomquist, R.G., 1994, Low-temperature geothermal resources of Washington: Washington Division of Geology and Earth Resources Open-file Report 94-11, 53 p.
Silver, P.G., and Valette-Silver, H.J., 1992, Detection of hydrothermal precursors to large northern California earthquakes: Science, v. 257, p. 1363–1368, doi: 10.1126/science.257.5075.1363.
Smith, J.G., Dehn, J., Hoblitt, R.P., LaHusen, R.G., Lowenstern, J.B., Moran, S.C., McClelland, L., McGee, K.A., Nathenson, M., Okubo, P.G., Pallister, J.S., Poland, M.P., Power, J.A., Schneider, D.J., and Sisson, T.W., 2009, this volume, Volcano monitoring, in Young, R., and Norby, L., Geological Monitoring: Boulder, Colorado, Geological Society of America, doi: 10.1130/2009.monitoring(12).
Sorey, M.L., 2000, Geothermal Development and changes in surficial features: Examples from the western United States: Proceedings of the World Geothermal Congress, p. 705–711.
Sorey, M.L., Nathenson, M., and Smith, C., 1983, Methods for assessing lot temperature geothermal resources, in Reed, M.J., ed., Assessment of Low-Temperature Geothermal Resources of the United States, 1982: U.S. Geological Survey Circular 892, p. 17–30.
U.S. Geological Survey, 2003, Hydrologic studies in Long Valley Caldera: http://lvo.wr.usgs.gov/HydroStudies.html, accessed March 18, 2008.
Varley, J.D., and Schullery, P., 1998, Yellowstone Fishes: Stackpole Books, Mechanicsburg, Pennsylvania, 154 p.
Vital Sign 1
Planer-Friedrich, B., Becker, J., Brimer, B., and Merkel, B.J., 2008, Low-cost aerial photography for high-resolution mapping of hydrothermal areas in Yellowstone National Park: International Journal of Remote Sensing, v. 29, no. 6, p. 1781–1794, doi: 10.1080/01431160701395237.
Shean, D., 2006, Norris Geyser Basin’s dynamic hydrothermal features-using historical aerial photographs to detect change: Yellowstone Science, v. 14, no. 4, p. 24–28.
Watson, F.G.R., Lockwood, R.E., Newman, W.B., Anderson, T.N., and Garrott, R.A., 2008, Development and comparison of Landsat radiometric and snowpack model inversion techniques for estimating geothermal heat flux: Remote Sensing of Environment, v. 112, p. 471–481, doi: 10.1016/j.rse.2007.05.010.
Vital Sign 2
Compton, R.R., 1962, Manual of fi eld geology: New York, John Wiley, 378 p.
Planer-Friedrich, B., Becker, J., Brimer, B., and Merkel, B.J., 2008, Low-cost aerial photography for high-resolution mapping of hydrothermal areas in Yellowstone National Park: International Journal of Remote Sensing, v. 29, no. 6, p. 1781–1794, doi: 10.1080/01431160701395237.
White, D.E., 1969, Rapid heat-fl ow surveying of geothermal areas utilizing individual snowfalls as calorimeters: Journal of Geophysical Research, v. 74, no. 22, p. 5191–5201, doi: 10.1029/JB074i022p05191.
Vital Sign 3
Carter, A.J., Ramsey, M.S., Belousov, A.B., Wessels, R.L., and Dehn, J., 2006, The January 2005 eruption of Bezymianny Volcano: Comparing ground and airborne thermal camera images to rapid response ASTER data: Eos (Transactions, American Geophysical Union) v. 86, no. 52, Fall Meeting Supplement, Abstract V31A–0604.
Ge, S., 1998, Estimation of groundwater velocity in localized fracture zones from well temperature profiles: Journal of Volcanology and Geothermal Research, v. 84, no. 12, p. 93–101, doi: 10.1016/S0377-0273(98) 00032-8.
Hook, S.J., Myers, J.J., Thome, K.J., Fitzgerald, M., and Kahle, A.B., 2001, The MODIS/ASTER airborne simulator (MASTER)—A new instrument for earth science studies: Remote Sensing of Environment, v. 76, no. 1, p. 93–102, doi: 10.1016/S0034 -4257(00)00195-4.
Kahle, A.B., 1987, Surface emittance, temperature, and thermal inertia derived from thermal infrared multispectral scanner (TIMS) data for Death Valley, California: Geophysics, v. 52, no. 7, p. 858–874, doi: 10.1190/1.1442357.
Neale, C.M.U., Jaworowski, C., Sivarajan, S., Akasheh, O., Cardenas, B., and Heasler, H.P., 2008, Monitoring thermal springs in Yellowstone National Park using high-resolution imagery [abs.]: Utah State University, Fourth Annual Spring Runoff Conference.
Pergola, N., Marchese, F., and Tramutolic, V., 2004, Automated detection of thermal features of active volcanoes by means of infrared AVHRR records: Remote Sensing of Environment, v. 93, p. 311–327, doi: 10.1016/j.rse.2004.07.010.
Pieri, D., and Abrams, M., 2004, ASTER watches the world’s volcanoes: a new paradigm for volcanological observations from orbit: Journal of Volcanology and Geothermal Research, v. 135, p. 13–28, doi: 10.1016/j.jvolgeores.2003.12.018.
Vaughan, R.G., Hook, S.J., Ramsey, M.S., Realmuto, V.J., and Schneider, D.J., 2005, Remotely monitoring volcanic activity at Mount St. Helens with thermal infrared data: Geological Society of America Abstracts with Programs, v. 37, no. 7, p. 108.
Vaughan, R.G., Hook, S.J., and Davies, A.G., 2006, Spaceborne thermal infrared measurements of volcanic thermal features: Eos (Transactions, American Geophysical Union) Fall Meeting Supplement, v. 87, p. 52.
Watson, F.G.R., Lockwood, R.E., Newman, W.B., Anderson, T.N., and Garrott, R.A., 2008, Development and comparison of Landsat radiometric and snowpack model inversion techniques for estimating geothermal heat flux: Remote Sensing of Environment, v. 112, p. 471–481, doi: 10.1016/j.rse. 2007.05.010.
Ziagos, J.P., and Blackwell, D.D., 1981, A model for the effect of horizontal fluid flow in a thin aquifer on temperature-depth profiles: Geothermal Resources Council Transactions, v. 5, p. 221–224.
Vital Sign 4
Buchanan, T.J., and Somers, W.P., 1969, Discharge measurements at gaging stations: U.S. Geological Survey Techniques of Water-Resources Investigations, Book 3, Chapter A8, 65 p.
International Organization for Standardization, 1983, Measurement of liquid flow in open channels: International Organization for Standardization Handbook 16, 518 p.
Nolan, K.M., and Shields, R.R., 2000, Measurement of stream discharge by wading: Water Resources Investigations Report 00-4036. Rantz, S.E., 1982, Measurement and computation of streamflow, volumes I and II: U.S. Geological Survey Water Supply Paper 2175, 631 p.
Sauer, V.B., and Meyer, R.W., 1992, Determination of error in individual discharge measurements: U.S. Geological Survey Open-fi le report 92-144.
Vital Sign 5
Ellis, A.J., and Mahon, W.A.J., 1977, Chemistry and geothermal systems: New York, Academic Press, 392 p.
Foley, D., and Street, L.V., 1988, Hydrothermal systems of the Wood River Area, Idaho, in Link, P.K., and Hackett, W.R., eds., Guidebook to the Geology of Central and Southern Idaho: Idaho Geological Survey Bulletin 27, p. 109–126.
Foley, D., 2006, Dating Castle Geyser: preliminary results and broad speculations on the geologic development of geysers and hydrothermal systems in Yellowstone National Park, Wyoming, USA: Geothermal Resources Council Transactions, v. 30, p. 413–417.
Fournier, R.O., Weltman, U., Counce, D., White, L.D., and Janik, C.J., 2002, Results of weekly chemical and isotopic monitoring of selected springs in Norris Geyser Basin, Yellowstone National Park during June-September, 1995: U.S. Geological Survey Open-File Report 02-344, 50 p.
Hem, J.D., 1985, Study and interpretation of the chemical characteristics of natural water: U.S. Geological Survey Water-Supply Paper 2254, 264 p.
Henley, R.W., and Ellis, A.J., 1983, Geothermal systems ancient and modern: a geochemical review: Earth-Science Reviews, v. 19, p. 1–50, doi: 10.1016/0012-8252(83)90075-2.
Kharaka, Y.K., Mariner, R.H., Bullen, T.D., Kennedy, B.M., and Sturchio, N.C., 1991, Geochemical investigations of hydraulic connections between the Corwin Springs Known Geothermal Resources Area and adjacent parts of Yellowstone National Park, in Sorey, M.L., ed., Effects of potential geothermal development in the Corwin Springs Known Geothermal Resources Area, Montana, on the thermal features of Yellowstone National Park: U.S. Geological Survey Water Resources Investigations Report 91-4052, p. F-1–F-38.
Mazor, E., 1991, Applied chemical and isotopic groundwater hydrology: New York, Halstead Press, 274 p.
Nicholson, K., 1993, Geothermal fl uids—Chemistry and exploration techniques: New York, Springer Verlag, 263 p.
Advanced Monitoring Techniques that Measure Multiple Vital Signs
Friedman, I., and Norton, D.R., 2007, Is Yellowstone losing its steam—Chloride flux out of Yellowstone National Park, in Morgan, L.A., ed., Integrated geoscience studies in the greater Yellowstone area—Volcanic, tectonic, and hydrothermal processes in the Yellowstone geoecosystem: U.S. Geological Survey Professional Paper 1717, p. 271–298.
Hurwitz, S., Lowenstern, J.B., and Heasler, H., 2007, Spatial and temporal geochemical trends in the hydrothermal system of Yellowstone National Park: Inferences from river solute fluxes: Journal of Volcanology and Geothermal Research, v. 162, p. 149–171, doi: 10.1016/j.jvolgeores.2007.01.003.
Kieffer, S.W., 1984, Seismicity at Old Faithful geyser: An isolated source of geothermal noise and possible analogue of volcanic seismicity: Journal of Volcanology and Geothermal Research, v. 22, p. 59–95, doi: 10.1016/0377-0273(84)90035-0.
Mariner, R.H., Presser, T.S., Evans, W.C., and Pringle, M.K.W., 1990, Discharge rates of fluid and heat by thermal springs of the Cascade Range, Washington, Oregon and northern California: Journal of Geophysical Research, v. 95, p. 19517–19531, doi: 10.1029/JB095iB12p19517.
Marler, G.D., 1964, Effects of the Hebgen Lake earthquake of August 17, 1959, on the hot springs of the Firehole Geyser basins, Yellowstone National Park: U.S. Geological Survey Professional Paper 0435, p. 185–197.
National Institute for Occupational Safety and Health (NIOSH), 1981, Occupational Health Guidelines for Chemical Hazards, DHHS (NIOSH) Publication No. 81–123, http://www.cdc.gov/niosh/81-123.html.
Sorey, M.L., 2000, Geothermal development and changes in surficial features: examples from the western United States: Proceedings of the World Geothermal Congress, p. 705–711.
Geothermal Monitoring Study Design
Cole, D.R., 1982, Tracing fl uid sources in the East Shore Area, Utah: Ground Water, v. 20, no. 5, p. 586–593, doi: 10.1111/j.1745-6584.1982. tb01374.x.
Ingebritsen, S.E., Galloway, D.L., Colvard, E.M., Sorey, M.L., and Mariner, R.H., 2001, Time-variation of hydrothermal discharge at selected sites in the western United States: implications for monitoring: Journal of Volcanology and Geothermal Research, v. 111, p. 1–23, doi: 10.1016/S0377-0273 (01)00207-4.
Jarvis, T.W., 1986, Regional hydrogeology of the Paleozoic Aquifer System, southeastern Bighorn Basin, Wyoming, with an User Impact Analysis on Hot Springs State Park [M.S. Thesis]: University of Wyoming at Laramie, 224 p.
Sorey, M.L., 1991, Effects of potential geothermal development in the Corwin Springs Known Geothermal Resources Area, Montana, on the thermal features of Yellowstone National Park: U.S. Geological Survey Water- Resources Investigations Report 91-4052, p. F1–F38.
Spencer, S.A., 1986, Groundwater movement in the Paleozioc rocks and impact of Petroleum Production on Water Levels in the Southwestern Bighorn Basin, Wyoming [M.S. Thesis]: University of Wyoming at Laramie, 165 p.
Case Study I
Breckenridge, R.S., and Hinckley, B.S., 1978, Thermal springs of Wyoming: Geological Survey of Wyoming, Bulletin 60, 104 p.
Buelow, K.L., Heasler, H.P., and Hinckley, B.S., 1986, Geothermal resources of the southern Powder River Basin, Wyoming: Geological Survey of Wyoming, Report of Investigations, no. 36, 32 p.
Heasler, H.P., 1982, The Cody hydrothermal system: Wyoming Geological Association Guidebook, 33rd Annual Field Conference, p. 163–174.
Heasler, H.P., and Hinckley, B.S., 1985, Geothermal resources of the Bighorn Basin, Wyoming: Geological Survey of Wyoming, Report of Investigations, no. 29, 28 p.
Heasler, H.P., Hinckley, B.S., Buelow, K.L., Spencer, S.A., and Decker, E.R., 1983, Geothermal resource map of Wyoming: National Oceanic and Atmospheric Association (publishers), available from the Geological Survey of Wyoming, P.O. Box 3008, Laramie, Wyoming, scale 1:500,000.
Hinckley, B.S., and Heasler, H.P., 1984, Geothermal resources of the Laramie, Hanna, and Shirley Basins, Wyoming: Geological Survey of Wyoming, Report of Investigations, no. 26, 26 p.
Hinckley, B.S., and Heasler, H.P., 1987, Geothermal resources of the Wind River Basin, Wyoming: Geological Survey of Wyoming, Report of Investigations, no. 38, 30 p.
Hinckley, B.S., Heasler, H.P., and King, J.K., 1982, The Thermopolis Hydrothermal System with an analysis of Hot Springs State Park: Geological Survey of Wyoming, Preliminary Report, no. 20, 42 p.
Jarvis, T.W., 1986, Regional hydrogeology of the Paleozoic Aquifer System, southeastern Bighorn Basin, Wyoming, with an User Impact Analysis on Hot Springs State Park [M.S. Thesis]: University of Wyoming at Laramie, 224 p.
Spencer, S.A., 1986, Groundwater movement in the Paleozioc rocks and impact of Petroleum Production on Water Levels in the Southwestern Bighorn Basin, Wyoming [M.S. Thesis]: University of Wyoming at Laramie, 165 p.
Case Study II
Allen, E.T., and Day, A.L., 1935, Hot springs of the Yellowstone National Park: Carnegie Institution of Washington Publication 466, 525 p.
Ball, J.W., McCleskey, R.B., Nordstrom, D.K., Holloway, J.M., and Verplanck, P.L., 2002, Water-chemistry data for selected springs, geysers, and streams in Yellowstone National Park, Wyoming, 1999–2000: U.S. Geological Survey Open-File Report 02-382, 112 p.
Ball, J.W., Nordstrom, D.K., Cunningham, K.M., Schoonen, M.A., Xu, Y., and DeMonge, J.M., 1998a, Water-chemistry and on-site sulfur-speciation data for selected springs in Yellowstone National Park, Wyoming, 1994–1995: U.S. Geological Survey Open-File Report 98–574, 35 p. [Revised and reprinted, April 2001.]
Ball, J.W., Nordstrom, D.K., Jenne, E.A., and Vivit, D.V., 1998b, Chemical analyses of hot springs, pools, geysers, and surface waters from Yellowstone National Park, Wyoming, and vicinity, 1974–1975: U.S. Geological Survey Open-File Report 98-182, 45 p.
Ball, J.W., Nordstrom, D.K., McCleskey, R.B., Schoonen, M.A., and Xu, Y., 2001, Water- chemistry and on-site sulfur-speciation data for selected springs in Yellowstone National Park, Wyoming, 1996–1998: U.S. Geological Survey Open-File Report 01-49.
Christiansen, R.L., 1975, Geologic Map of the Norris Junction quadrangle, Yellowstone National Park, Wyoming: U.S. Geological Survey Geologic Quadrangle Map GQ-1193, scale 1:62,500.
Fenner, C.N., 1936, Bore-hole investigations in Yellowstone National Park: The Journal of Geology, v. 44, p. 225–315.
Fournier, R.O., White, D.E., and Truesdell, A.H., 1976, Convective heat flow in Yellowstone National Park, in United Nations Symposium on the Development and Utilization of Geothermal Resources, Pisa, 1970: Geothermics Special Issue 2, v. 2, pt. 1, p. 529–535.
Fournier, R.O., Thompson, J.M., Cunningham, C.G., and Hutchinson, R.A., 1991, Conditions leading to a recent small hydrothermal explosion at Yellowstone National Park: Geological Society of America Bulletin, v. 103, p. 1114–1120, doi: 10.1130/0016-7606(1991)103<1114:CLTARS>2.3. CO;2.
Fournier, R.O., Weltman, U., Counce, D., White, L.D., and Janik, C.J., 2002, Results of weekly chemical and isotopic monitoring of selected springs in Norris Geyser Basin, Yellowstone National Park during June-September 1995: U.S. Geological Survey Open-File Report 02-344, 50 p.
Friedman, I., and Norton, D.R., 2000, Data used for calculating chloride flux out of Yellowstone National Park for the water years 1983–1999: U.S. Geological Survey Open-File Report OF 00-0194, 48 p.
Gooch, F.A., and Whitefi eld, J.E., 1888, Analyses of waters of the Yellowstone National Park, with an account of the methods of analyses employed: U.S. Geological Survey Bulletin 47, 84 p.
Hague, A., 1911, Origin of the thermal waters in Yellowstone National Park: Geological Society of America Bulletin, v. 22, p. 102–122.
Hardy, C.C., 2005, Characterizing thermal features from multi-spectral remote sensing data using dynamic calibration procedures [Ph.D. dissertation]: University of Montana at Missoula, 153 p.
Kharaka, Y.K., Marnier, R.H., Bullen, T.D., Kennedy, B.M., and Sturchio, N.C., 1991, Geochemical investigations of hydraulic connections between Corwin Springs known geothermal resources area and adjacent parts of Yellowstone National Park, in Sorey, M.L., ed., Effects of potential geothermal development in the Corwin Springs known geothermal resources area, Montana on the thermal features of Yellowstone National Park: U.S. Geological Survey Water Resources Investigations Report 91-4052, p. F1–F38.
Richmond, G.M., and Waldrop, H.A., 1975, Surfi cial geologic map of the Norris Junction quadrangle, Yellowstone National Park, Wyoming: U.S. Geological Survey Miscellaneous Investigations Map I-650, scale 1:62,500.
White, D.E., 1957, Thermal waters of volcanic origin: Geological Society of America Bulletin, v. 68, p. 1637–1657, doi: 10.1130/0016-7606(1957)68 [1637:TWOVO]2.0.CO;2.
White, D.E., Fournier, R.O., Muffl er, L.J.P., and Truesdell, A.H., 1975, Physical results from research drilling in thermal areas of Yellowstone National Park Wyoming: U.S. Geological Survey Professional Paper 892, 77 p.
White, D.E., Hutchinson, R.A., and Keith, T.E.C., 1988, The geology and remarkable chemical activity of Norris Geyser Basin, Yellowstone National Park, Wyoming: U.S. Geological Survey Professional Paper 1456, 84 p.
ANNOTATED BIBLIOGRAPHY
Blackwell, D.D., Negraru, P.T., and Richards, M.C., 2006, Assessment of the Enhanced Geothermal System Resource Base of the United States: Natural Resources Research, v. 15, no. 4, p. 283–308, doi: 10.1007/s11053-007-9028-7. (This paper describes the nature of enhanced geothermal resources and subsurface thermal conditions in the United States. Although enhanced geothermal resources are not part of the monitoring efforts described in this chapter, the maps included by the authors are helpful in identifying areas of the United States that are warmer at depth.)
Wohletz, K., and Heiken, G., 1992, Volcanology and Geothermal Energy: Berkeley, California, University of California Press, 432 p. (A thorough discussion of different types of volcanoes and their associated geothermal systems. Includes suggestions for field work in volcanic regions, and extensive descriptions of types and locations of hydrothermal systems.)
GEOTHERMAL WEB SITES OF INTEREST
http://www1.eere.energy.gov/geothermal/ (U.S. Department of Energy geothermal home page.)
http://www.osti.gov/geothermal/servlets/purl/6737326-YY5cwq/6737326.pdf (Thermal springs of United States [(Berry et al., 1980] in PDF.)
http://www.ngdc.noaa.gov/nndc/servlet/ShowDatasets?dataset=100006&sea rch_look=1&display_look=1 (Thermal springs of United States [Berry et al., 1980] in searchable format.)
http://www.unr.edu/geothermal/datalist.html (GIS layers of data for the Great Basin.)
http://geothermal.inl.gov/ (Maps of geothermal resources in western states.)
http://water.usgs.gov/nrp/proj.bib/mariner.html (Publications on geothermal—lots of good U.S. Geological Survey references.)
http://www.uwiseismic.com/Documents/Presentations/SRU%20Geothermal%20Monitoring%20Program_fi les/frame.htm#slide0001.htm (University of West Indies; lists chemical constituents being sampled.)
http://www.ngdc.noaa.gov/seg/geotherm.shtml (Thermal springs of United States list.)
http://www.yourownpower.com/Power/ (Chena power plant description.)
http://pubs.usgs.gov/twri/twri5-a1/html/pdf.html (Determination of inorganic substances in water and fluvial sediments.)
http://pubs.usgs.gov/of/1995/of95-689/ (Digital maps of low and moderate temperature resources in Pacific Northwest.)
http://www1.eere.energy.gov/geothermal/glossary.html (A geothermal glossary.)
http://www.geothermal.org/powerpoint07_workshop.html (Geothermal Resources Council 2007 Annual Meeting PowerPoint Presentations Geophysical Techniques in Geothermal Exploration Workshop September 28–29; much valuable data. The Geothermal Resources Council also has much additional data.)
http://www.unr.edu/geothermal/centerinfo.html (The Great Basin Center for Geothermal Energy at the University of Nevada–Reno provides geoscientific data on the Great Basin.)
http://www.smu.edu/geothermal (Thermal data for the United States.)
http://geoheat.oit.edu (Data on low- and moderate-temperature resource uses throughout the United States and beyond.)
http://www.environmentwaikato.govt.nz/enviroinfo/redi/index.htm (Geothermal and environmental monitoring data for the Waikato area, New Zealand.)