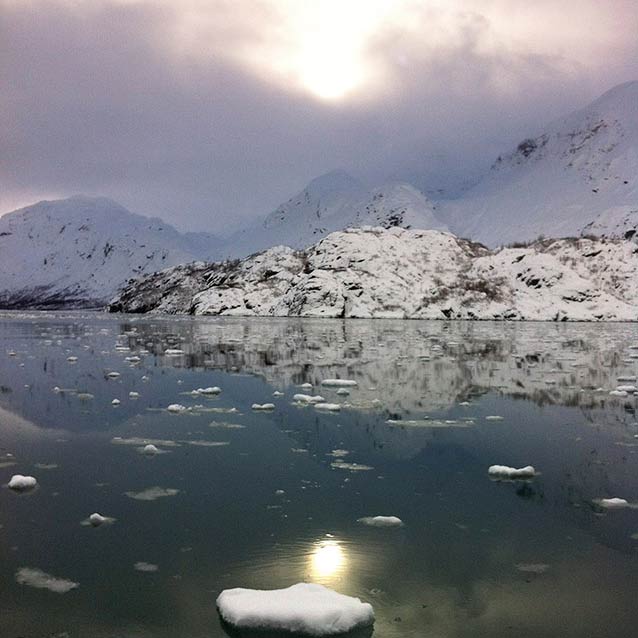
NPS Photo
Since the start of the Industrial Revolution in the late eighteenth century, mankind has emitted a large volume of carbon dioxide (CO2) into the atmosphere, primarily from the burning of fossil fuel (Sabine et al. 2004). This CO2 is ultimately partitioned among the atmosphere, terrestrial, and marine ecosystems. When the marine ecosystem takes up CO2 from the atmosphere, it causes the pH of the water to decrease, making it more acidic over time, and can lead to negative effects on some organisms within these waters. Approximately 46 percent of anthropogenic, or man-made, CO2 remains in the atmosphere, while about 28 percent is taken up by the terrestrial biosphere, and the remaining 26 percent is absorbed by the ocean (Sabine et al. 2004). This increase in oceanic CO2 has led to an increase in dissolved inorganic carbon (DIC) concentrations and a reduction in global ocean pH of approximately 0.1 units (Feely et al. 2004). However, the uptake of atmospheric CO2 is not the only climate-induced phenomenon that can lead to a reduction of marine pH. The addition of freshwater, such as glacial melt, can also impact seawater chemistry and contribute to decreased pH.
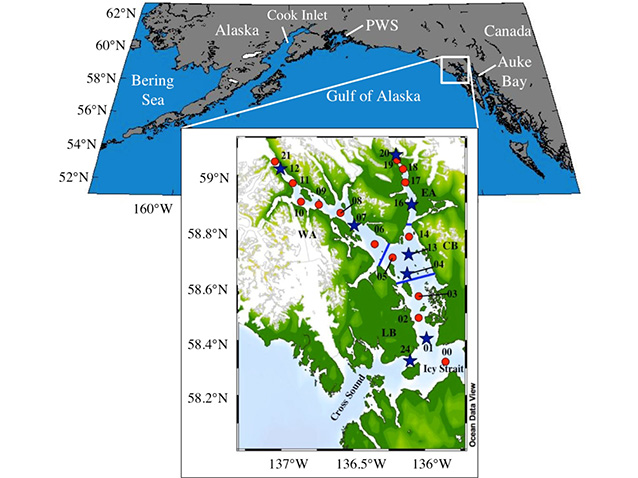
Although Alaska’s coasts contain more than 200 major fjords, few have been studied in detail (Etherington et al. 2007) and several receive large volumes of glacial runoff. Glacier Bay, within Glacier Bay National Park and Preserve, lies along the eastern coast of the Gulf of Alaska in Southeast Alaska (Figure 2) and is an example of a pristine tidewater glacial fjord ecosystem. It was once occupied by one large icefield, aptly named the Glacier Bay Icefield, which has experienced rapid deglaciation since the end of the Little Ice Age around AD1770 (Johnson et al. 2013). As a result of this deglaciation, Glacier Bay is now surrounded by a number of tidewater and alpine glaciers. The bay has experienced one of the most rapid deglaciations on record (Pfeffer et al. 2000), increasing the amount of freshwater runoff into the marine ecosystem and affecting the chemistry, biology, and flow dynamics of the bay (Hill et al. 2009).
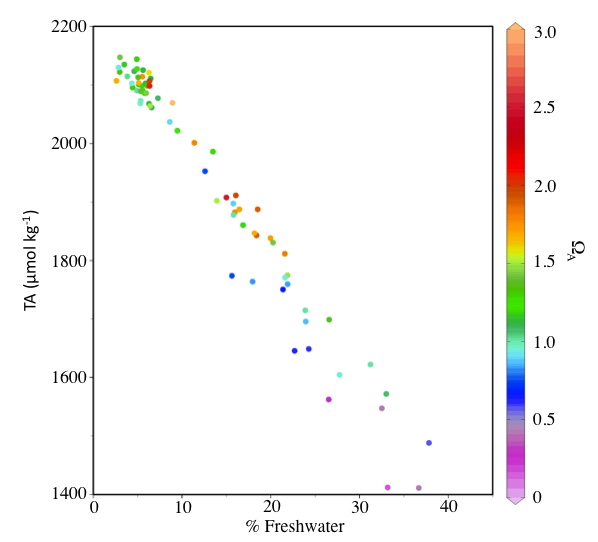
Freshwater, including glacial melt, has lower alkalinity than oceanic water. Alkalinity is a measure of the capacity of seawater to neutralize or “buffer” acids. When freshwater enters seawater, it dilutes the alkalinity in the seawater, making it less able to buffer against decreases in pH. When glacial melt enters the marine waters of Glacier Bay, it dilutes alkalinity, allowing the uptake of atmospheric CO2 by the surface waters to more easily cause a decrease in pH, contributing to ocean acidification. The Glacier Bay marine ecosystem, along with similar fjord systems around the Gulf of Alaska, is highly influenced by freshwater runoff. Therefore, alkalinity must be taken into account when analyzing Glacier Bay’s susceptibility to ocean acidification.
What is Ocean Acidification?
Ocean acidification (OA) refers to the increase in ocean acidity (decrease in ocean pH), typically caused by the dissolution of atmospheric CO2 gas into seawater. When CO2 is absorbed by seawater, chemical reactions occur that reduce seawater pH and calcium carbonate (CaCO3) ion concentration. These chemical reactions are termed ocean acidification. Because the pH scale is logarithmic, the roughly 0.1 pH unit decrease in ocean water during the Industrial Revolution translates into a roughly 30 percent increase in acidity (Frisch et al. 2015).
Other factors can exacerbate the severity and duration of OA events. Freshwater inputs, such as glacial melt, are low in alkalinity. These additions “dilute” seawater, reducing its capacity to buffer against the reductions in pH that are driven by the uptake of atmospheric CO2. In this way, ocean waters receiving lots of glacial melt are more susceptible than usual to OA.
The severity of OA is assessed using saturation states. Saturations states act as a numerical index to describe the water chemistry in terms of the current degree of OA. They are calculated with respect to calcium carbonate minerals, such as aragonite, which are used by many marine organisms to build shells and skeletons. When the saturation state is greater than 1.0, waters are considered supersaturated with respect to calcium carbonate minerals. This means there is an abundant supply of these minerals available for use by calcifying organisms such as pteropods (tiny planktonic snails), clams, and crabs. However, OA can cause parts of the ocean to become undersaturated with respect to these minerals (saturation states less than 1.0), which likely impacts the ability of some organisms to produce and maintain their shells. This may lead to profound changes in marine ecosystems. Conversely, when organisms undergo photosynthesis, or primary production, they take up CO2, the major constituent of DIC, from surface waters and can lead to an increase in surface water saturation states, mitigating some of the effects of reduced alkalinity.
Seasonal Ocean Acidification in Glacier Bay
We found that Glacier Bay waters experienced seasonal and regional ocean acidification events (i.e., times when saturations states were less than 1.0). During the spring, summer, and early fall seasons, primary production is at elevated levels, consuming DIC in the surface waters. This reduces DIC concentrations and results in increased saturations states. However, glacial melt is also higher during those same seasons. When glacial melt enters the surface waters it dilutes alkalinity and can lead to reduced saturation states.
Low-saturation states observed in Glacier Bay were well correlated with the timing of maximum glacial discharge events and were most prominent within the two arms where glacial influence was greatest. Figure 3 shows all surface samples collected between July 2011 and July 2012. It illustrates that the regions of the bay receiving the most freshwater input (the upper arms) also had the lowest alkalinity concentrations and saturation states. Saturation states reached a minimum of 0.40 at the surface during the summer of 2011. Saturation states increased from the upper to the lower bay as waters became less influenced by glacial runoff.
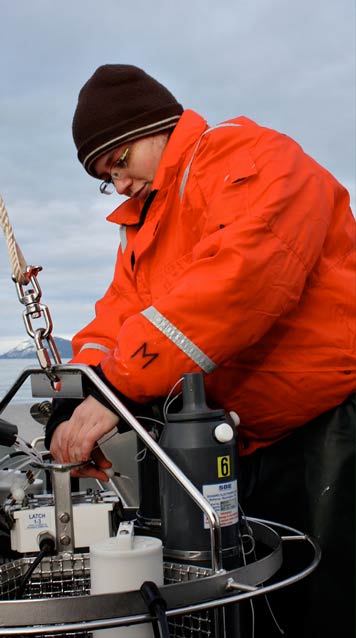
During the fall, all Glacier Bay surface waters had saturation states below 1.0. This may have been due to increased winds during fall that mixed DIC from depth back into the upper water column, while simultaneously enhancing atmospheric CO2 uptake by the surface waters. The bay remained relatively well mixed throughout the winter, with elevated concentrations of DIC and alkalinity as a result of low primary production, reduced glacial melt, and greater wind mixing.
Saturation states returned to supersaturated conditions (i.e., saturation states greater than 1.0) in the spring of 2012. During spring, increased primary production caused a reduction in DIC in the surface waters, initiating an increase in saturation states before glacial runoff peaked. Conditions in the upper arms became undersaturated once again during the summer, with increasing glacial runoff overwhelming any drawdown of DIC from primary production.
Impacts of Anthropogenic CO2
The uptake of anthropogenic CO2 has already lowered the average ocean pH by approximately 0.1 units, with a continued reduction of 0.1 to 0.5 units expected over the next 100 years (Feely et al. 2004). While most OA research to date has been conducted in the open ocean, few studies have focused on near-shore estuarine ecosystems (Figure 4). Unique coastal processes reduce salinity and alkalinity concentrations and dampen the buffering capacity of these waters, making them more susceptible to changes in pH than the open ocean (Miller et al. 2009). It is important to understand, however, that these waters also naturally have seasonally dynamic pH values that may not all directly reflect current anthropogenic influences.
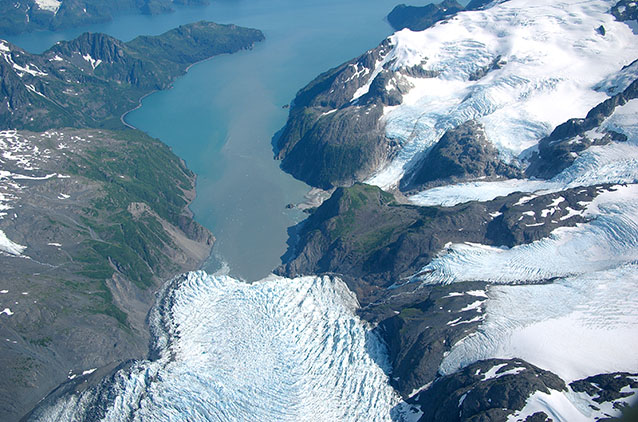
NPS Photo
Atmospheric CO2 is made up of emissions from natural as well as anthropogenic sources, such as the burning of fossil fuels. While we cannot directly quantify the amount of anthropogenic CO2 versus naturally occurring CO2 in Glacier Bay, its effects on dissolved carbon concentrations in the bay can be estimated. To estimate these anthropogenic effects on OA in Glacier Bay, about 45 moles kg−1 were removed from the measured DIC values to represent pre-industrial conditions, while all remaining variables remained as observed (Mathis and Questel 2013). Saturation states were recalculated for each season using the seasonal pre-industrial DIC values. Although all saturation states increased with the removal of the anthropogenic CO2 signal, the effect of anthropogenic CO2 was most notable during the seasons with the lowest surface saturation states (i.e., summer and fall). During the summer seasons, the only samples that remained undersaturated after removing the anthropogenic signal were those of surface waters within the arms of the bay where glacial influence was greatest. While saturation states during the fall indicated that all surface waters, as well as several samples from deeper depths, were undersaturated, upon removal of the anthropogenic CO2 signal, the fall samples from all depths became supersaturated. This simple approximation demonstrates the impact that anthropogenic CO2 has on marine systems like Glacier Bay.
Potential Future Implications
As atmospheric CO2 concentrations continue to rise, the anthropogenic signal in seawater will also increase. Wanninkhof et al. (2013) showed that the partial pressure of CO2 (pCO2) is increasing in the North Pacific by about 1.5 ppm per year, or about 75 ppm every 50 years. Using this rate of increasing pCO2 along with data from our discrete measurements, we calculated that saturation states with respect to aragonite have the potential to decrease by an average of 0.16 throughout surface waters of Glacier Bay in the next 50 years, with the largest effects seen during the spring season. We also found that if atmospheric CO2 trends continue at their current rate, the surface waters of the bay will become perennially undersaturated in aragonite in approximately 150 years. However, because the rate of CO2 accumulation in the ocean is also increasing, it is likely that this is a conservative estimate and does not take into account the changes in pCO2 due to potential seawater temperature changes in the bay as the climate warms. Additionally, this estimate does not include potential acidifying affects of increased freshwater and glacial inputs due to rising atmospheric temperatures, which will also increase pCO2, and lower total alkalinity concentrations compared to pre-industrial values.
Summary
Glacier Bay experienced seasonal and regional ocean acidification events during 2011-2012, and we belive this is the usual pattern. Areas where OA conditions were most severe and prolonged correlated with regions of the bay that experienced the greatest degree of glacial influence (i.e., the east and west arms). Saturation states were lowest in the upper east and west arms during the summer seasons, and all surface waters were found to be undersaturated during the spring of 2011. Saturation states rebounded to greater than 1.0 across the bay during the winter, and waters remained supersaturated through the spring season as primary production reduced DIC concentrations in the surface waters.
When the anthropogenic CO2 signal was removed from current seasonal conditions, saturation states increased and, in most cases, waters became supersaturated with respect to calcium carbonate minerals. However, waters nearest the glacial outflows in the upper arms remained undersaturated due to the influence of low-alkalinity meltwater. Estimations of future OA conditions show the potential for saturation states to decrease by 0.16 units over the next 50 years. Projecting these estimates farther into the future, we believe that surface waters throughout Glacier Bay have the potential to become undersaturated year-round within the next 150-200 years. However, these estimates are based on current DIC and alkalinity concentrations, as well as current rates of glacial runoff. More extensive study is necessary to understand how continued glacial retreat will impact future DIC and alkalinity concentrations in Glacier Bay.
Acknowledgements
The author wishes to thank Lewis Sharman, Seth Danielson, Brendan Moynahan, Christopher Sergeant, and the employees of Glacier Bay National Park and Preserve. Funding for this research was provided by the National Park Service’s Southeast Alaska Network Inventory and Monitoring Program and the University of Alaska-Fairbanks.
Further information available at:
NOAA-PMEL Ocean Acidification: http://oceanacidification.noaa.gov/
References
Etherington, L., P. Hooge, E. Hooge, and D. Hill. 2007.
Oceanography of Glacier Bay, Alaska: implications for biological patterns in a glacial fjord estuary. Estuaries and Coasts 30(6): 927–944.
Feely, R., C. Sabine, K. Lee, W. Berelson, J. Kleypas, V. Fabry, and F. Millero. 2004.
Impact of anthropogenic CO2 on the CaCO3 system in the oceans. Science 305: 362–366. doi:10.1126/science.1097329.
Frisch, L., J. Mathis, N. Kettle, and S. Trainor. 2015.
Gauging perceptions of ocean acidification in Alaska. Marine Policy 53: 101–110.
Hill, D., S. Ciavola, L. Etherington, and M. Klaar. 2009.
Estimation of freshwater runoff into Glacier Bay, Alaska and incorporation into a tidal circulation model. Estuarine, Coastal and Shelf Science 82: 95–107.
Johnson, A., C. Larsen, N. Murphy, A. Arendt, and S. Zirnheld. 2013.
Mass balance in the Glacier Bay area of Alaska, USA, and British Columbia, Canada, 1995-2011, using airborne laser altimetry. Journal of Glaciology 59: 632–648.
Mathis, J. and J. Questel. 2013.
Assessing seasonal changes in carbonate parameters across small spatial gradients in the Northeastern Chukchi Sea. Continental Shelf Research 67: 42–51. doi:10.1016/j.csr.2013.04.041
Miller, A., A. Reynolds, C. Sobrino, G. Riedel. 2009.
Shellfish face uncertain future in high CO2 world: influence of acidification on oyster larvae calcification and growth in estuaries. PloS one 4: e5661 doi: http://journals.plos.org/plosone/article?id=10.1371/journal.pone.0005661
Pfeffer, W., J. Cohn, M. Meier, and R. Krimmel. 2000.
Alaskan glacier beats a rapid retreat. Eos Transactions American Geophysical Union 81(48): 577–584.
Reisdorph, S. and J. Mathis. 2014.
The dynamic controls on carbonate mineral saturation states and ocean acidification in a glacially dominated estuary. Estuarine, Coastal and Shelf Science 144: 8–18.
Sabine, C., et al. 2004.
The oceanic sink for anthropogenic CO2. Science 305: 367–371. doi:10.1126/science.1097403
Wanninkhof, R., et al. 2013.
Global ocean carbon uptake: magnitude, variability and trends. Biogeosciences 10: 1983–2000.
Part of a series of articles titled Alaska Park Science - Volume 15 Issue 1: Coastal Research Science in Alaska's National Parks.
Last updated: October 26, 2021